the Creative Commons Attribution 4.0 License.
the Creative Commons Attribution 4.0 License.
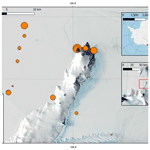
First evidence of microplastics in Antarctic snow
Alex R. Aves
Laura E. Revell
Sally Gaw
Helena Ruffell
Alex Schuddeboom
Ngaire E. Wotherspoon
Michelle LaRue
Adrian J. McDonald
In recent years, airborne microplastics have been identified in a range of remote environments. However, data throughout the Southern Hemisphere, in particular Antarctica, are largely absent to date. We collected snow samples from 19 sites across the Ross Island region of Antarctica. Suspected microplastic particles were isolated and their composition confirmed using micro-Fourier transform infrared spectroscopy (µFTIR). We identified microplastics in all Antarctic snow samples at an average concentration of 29 particles L−1, with fibres the most common morphotype and polyethylene terephthalate (PET) the most common polymer. To investigate sources, backward air mass trajectories were run from the time of sampling. These indicate potential long-range transportation of up to 6000 km, assuming a residence time of 6.5 d. Local sources were also identified as potential inputs into the environment as the polymers identified were consistent with those used in clothing and equipment from nearby research stations. This study adds to the growing body of literature regarding microplastics as a ubiquitous airborne pollutant and establishes their presence in Antarctica.
- Article
(13582 KB) - Full-text XML
-
Supplement
(237 KB) - BibTeX
- EndNote
Over the last century plastics have become one of the most ubiquitous synthetic materials in the world due to their versatility and durability. Despite their longevity, plastics degrade over time to produce microplastics (plastic particles < 5 mm in diameter), and when present in the environment, they have the potential to cause significant ecological damage (MacLeod et al., 2021). Microplastics have negative effects on marine organisms (Wright et al., 2013) and act as vectors for persistent organic pollutants and other toxic substances (Mato et al., 2001; Rios et al., 2007), which are harmful to marine environments and organisms (Hermabessiere et al., 2017). Microplastics have been recognized as widespread pollutants in the marine environment (Ryan, 2015) and are known to be damaging to terrestrial ecosystems (de Souza Machado et al., 2018), while their small size and relatively low density also allow them to become airborne and transported over large distances (Evangeliou et al., 2020).
Airborne microplastics have been identified in atmospheric fallout in a range of urban (Dris et al., 2015, 2016; Cai et al., 2017; Klein and Fischer, 2019; Knobloch et al., 2021) and remote regions worldwide (Allen et al., 2019; Bergmann et al., 2019; Brahney et al., 2020). It is now understood that microplastics transition between marine environments, terrestrial environments and the atmosphere via the plastic cycle (Horton and Dixon, 2018; Brahney et al., 2021). This allows microplastics to reach locations far from anthropogenic sources, such as the Arctic (Bergmann et al., 2019), the Tibetan Plateau (Zhang et al., 2021), European alpine regions (Allen et al., 2019; Bergmann et al., 2019; Materić et al., 2020, 2021) and conservation areas across the continental United States (Brahney et al., 2020). Deposited microplastics may accelerate melting of the cryosphere when present on snow and ice in alpine or polar regions (Evangeliou et al., 2020). Microplastics may further influence climate by acting as cloud ice nuclei in the atmosphere (Ganguly and Ariya, 2019) and through their minor contribution to global radiative forcing (Revell et al., 2021).
With a few exceptions, such as lead pollution in the late 19th century (McConnell et al., 2014), Antarctica was generally thought to be largely untouched by humans until the early 20th century due to its inaccessibility, extreme environmental conditions and barriers such as the Antarctic Circumpolar Current (Tin et al., 2014; Gordon, 1971). While the human footprint has increased over the last century, Antarctica is still a place of peace and science and is thought of as the last remaining true wilderness on earth (Tin et al., 2016). Due to this, Antarctica can act as an indicator of physical, chemical and biological effects caused by anthropogenic stresses (Huiskes et al., 2006). Research on microplastics in the Antarctic has focused on the marine environment, where particles have been detected in deep sea sediments in the Weddell Sea (Van Cauwenberghe et al., 2013), marine sediments from the western Antarctic Peninsula (Reed et al., 2018) and the Ross Sea (Munari et al., 2017), south of the Polar Front (Cózar et al., 2014), and in the surface waters of the Southern Ocean and Antarctic Peninsula (Absher et al., 2019; Cincinelli et al., 2017; Isobe et al., 2017; Suaria et al., 2020; Waller et al., 2017; Lacerda et al., 2019). Microplastics were recently identified for the first time in a freshwater Antarctic Specially Protected Area (ASPA) on Livingston Island, which is used for long-term ecological monitoring due to its pristine nature and use as a reference for inland water research (González-Pleiter et al., 2020).
To date there is little information available regarding the presence of airborne microplastics in Antarctica. We collected freshly fallen snow samples from the Ross Island region of Antarctica in late 2019 and analysed them to quantify the presence and abundance of microplastics. Samples were collected close to two scientific research stations (Scott Base and McMurdo Station) and from 13 field sites up to 20 km from the research stations. We identified polymer composition using micro-Fourier transform infrared spectroscopy (µFTIR) and analysed air mass back trajectories to identify the potential origins of sampled air masses. Further, we catalogued the composition of field equipment to understand local polymer sources.
2.1 Field collection
Snow samples were collected in 500 mL stainless-steel bottles. A total of 19 samples were collected with 6 from locations near research stations and 13 from remote locations with minimal human disturbance (Fig. 1). Samples were collected using a stainless-steel scoop and stainless-steel funnel to fill each 500 mL bottle with snow from the top 2 cm of the surface. Samples were stored in a −25 ∘C freezer at Scott Base and were kept chilled with dry ice during transit to New Zealand. Upon arrival in New Zealand, samples were stored at −20 ∘C.
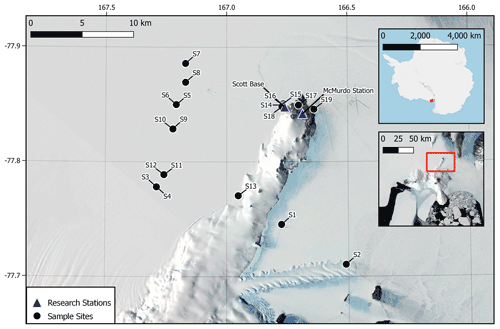
Figure 1Site locations for snow sample collections across the Ross Island region of Antarctica (S1–S19). Sample sites are marked in black, and locations of scientific research stations are shown by blue triangles. Some site markers correspond to two sampling sites due to the scale of the map. Map data sourced from Matsuoka et al. (2021).
2.2 Laboratory analysis of microplastics
Snow samples were thawed in the sealed sample bottles at room temperature for 24–48 h prior to analysis. Thawed samples were filtered through a glass apparatus attached to a vacuum using a cellulose nitrate membrane filter (Whatman nitrocellulose membrane, 50 mm diameter, 0.45 µm pore size). The volume of liquid (melted snow) was recorded at this step, before rinsing, to establish the volume of each individual sample bottle (Table A2). Approximately 10–20 mL of 70 % ethanol was used to rinse the filters, and a further 10–30 mL of 96 % ethanol was added to soak the filter for 10 min to prevent bacterial and viral growth for further biosecurity measures. The sides of the glassware were thoroughly rinsed with ultra-pure water (<18 MΩ) to dislodge any microplastics adhering to the walls of the filtering equipment, and samples were dried under vacuum.
The dried cellulose nitrate filter was transferred to a 250 mL glass beaker for a wet peroxide oxidation (WPO) digestion to remove organic material present on the filter. An Iron (Fe(II)) sulfate solution (0.05 M) was prepared by adding 7.5 g of FeSO4 ⋅ 7H2O (=278.02 g mol−1) to 500 mL of ultra-pure water and 3 mL of concentrated sulfuric acid (Fenton's reagent). This study followed similar methods to those outlined by the National Oceanic and Atmospheric Administration (NOAA) (Masura et al., 2015) for WPO digestion, with 20 mL of 30 % H2O2, 100 mL ultra-pure water and 5 mL of the Fe(II) solution added to the beaker with the filter. The solution was then left, with the beaker opening covered in aluminium foil, for 20 min before being stirred on a hot plate at 45 ∘C for 2–3 h with a magnetic stir bar. The magnetic stir bar had a white polytetrafluoroethylene (PTFE) coating, which was not identified in any field or blank samples.
The digested cellulose nitrate filter was rinsed with ultra-pure water to remove any attached material. The digested filtrate was then filtered under vacuum onto a Whatman glass fibre GF/C filter (47 mm diameter, 1.2 µm pore size), and all glassware was thoroughly rinsed with ultra-pure water. All reagents used were previously unopened and analytical grade, with blanks also undergoing identical analysis to control for contamination.The GF/C filter was then vacuum dried before being removed with tweezers into a closed petri dish and labelled accordingly for storage until analysis.
2.3 Visual characterization of microplastics and µFTIR analysis
Filter papers were initially screened using a Leica MZ125 stereomicroscope with 10× magnification for visual identification. Suspected plastic particles were identified according to characteristics related to the morphotype, shape, colour and the physical characteristics of each particle (Bridson et al., 2020). Suspected microplastics were characterized into four main morphotypes – fibres, films, fragments and beads. Colours were recorded for each suspected particle. Analysis was performed by the same individual to ensure consistency in identification and counts. Each filter was visually analysed three times. Due to limitations of visual identification techniques (Knobloch et al., 2021), dark colours were difficult to differentiate. Therefore throughout this study, “blue” includes blue, black and navy. It is recognized that, due to human error, an inability to transfer some particles due to their small size and brittleness, and the translucent and transparent nature of some microplastics, there are limitations to this method which are hard to avoid. This may lead to the underestimation of microplastics in this study. The smallest particle identified in this study was 44 µm (non-plastic), meaning particles less than this size were not accounted for due to analysis limitations.
All suspected microplastics were chemically identified by micro-Fourier transform infrared spectroscopy (µFTIR). This procedure followed pre-existing methods for characterizing microplastics (Primpke et al., 2018). A HYPERION 2000 µFTIR microscope (Bruker Optics), attached to a Vertex 70 (Bruker Optics) spectrometer, was used to analyse particles plated on a calcium fluoride (CaF2) disc (25 mm diameter), with a liquid-nitrogen-cooled mercury–cadmium–telluride (MCT) detector. Each particle was manually transferred onto the disc using tweezers and a drop of 96 % ethanol to aid in transfer. An optical overview image was recorded before infrared measurements were performed at 15× magnification. Scans were run in transmission mode (10 scans, 4 cm−1 resolution, spectral range of 4000–1000 cm−1) using OPUS 7.8 software. All spectra were baseline corrected, and the CO2 spectral range was excluded, saved and compared against the following Wiley spectral libraries (databases: industrial chemicals, pure organic compounds; organosilicons; polymers, Hummel defined basic; Sadtler acrylates and methacrylates; Sadtler fibres and textile chemicals; Sadtler fibres by microscope; Sadtler inorganics; Sadtler polymers and monomers (comprehensive); Sadtler polymers, Hummel; Sadtler standards (organic and polymeric compounds subset); Sigma-Aldrich library of FT-IR spectra). Particles returning a hit quality index (HQI) of > 70 % against the library reference spectra were accepted as microplastics. Those < 70 % that exhibited plastic characteristics from visual screening and possessed similar µFTIR spectra were analysed further by the authors using Wiley peak picking tools to identify characteristic peaks of plastic polymer types (Kroon et al., 2018).These particles matching characteristic spectral peaks were included in results. Due to the environmental degradation that sampled particles have been subjected to, as well as the limitations of spectral libraries due to the use of high standard polymers, the visual inspection of spectra is an essential step in identification of environmental microplastic analysis (De Frond et al., 2021; Shim et al., 2017).
2.4 Quality control
2.4.1 Field sampling
Each sampling site was selected ensuring the presence of fresh snow with no visible contamination or movement in the collection area. Non-plastic sampling equipment was used to avoid contamination, and all equipment was rinsed in nearby snow prior to each sample collection. Leather gloves were worn by the sample collectors, and the sample site was selected upwind from any human movement to minimize contamination by the samplers. The lid of each sampling bottle was held without the inside being touched during sample collection to avoid human contamination. Bottles were stored upright and kept in a cool box in snow during transportation.
2.4.2 Controls and blanks
Two field controls were collected during sample collection: one alongside a remote field site sample and one alongside a research station sample. The two field control bottles were left open during snow sampling and filled with ultra-pure water when returned to the laboratory. They subsequently underwent the same laboratory methods as all other samples. Two method controls were prepared in stainless-steel bottles identical to the sampling bottles used for collection and were filled with ultra-pure water. These were stored in the laboratory freezer in New Zealand during sampling and underwent identical laboratory procedures as the samples to identify potential sources of contamination from the sampling bottles. For blank corrections we followed the methodology highlighted in Brander et al. (2020) and used in Vandermeersch et al. (2015), whereby particles matching the identical characteristics to those found in the blanks (laboratory and field blanks) were omitted from further analysis, and total daily laboratory blank findings were subtracted from the corresponding samples (Table S1). Daily laboratory blanks were analysed using the same procedure as all samples with 500 mL of ultra-pure water. The laboratory blanks were analysed to compare results for each individual date and accounted for in the data for the samples filtered on the corresponding days. Particles found in field samples with identical characteristics to those found in blanks were discarded and excluded from the results.
2.4.3 Laboratory analysis
Method recovery tests consisted of two 500 mL samples of ultra-pure water spiked with five polyethylene (PE) beads and seven polymethyl methacrylate (PMMA) fibres sized between 500 and 2000 µm. The spiked samples were analysed identically to the field samples to measure recovery rates of the spiked samples. Filtration and laboratory procedures were performed in a Labrocare fume hood cabinet to limit contamination by airborne microplastics. Glassware was cleaned inside the fume hood three times with ultra-pure water and once with acetone. Aluminium foil was used to cover glassware openings to minimize contamination by airborne microplastics. Non-synthetic (wool and cotton) clothing was worn during the laboratory and analysis process. Surfaces were sprayed and wiped down with 70 % ethanol prior to microscopy and analysis work, with filters remaining covered except when manual extraction of particles occurred.
2.5 Clothing composition
National Antarctic programmes provide essential clothing and field gear to staff and scientists. Some of the gear provided is mandatory whenever undertaking fieldwork or travelling outside of bases. The composition of field gear provided by the New Zealand National Antarctic programme (including base layers, mid-layers, outer layers, shoes, boot liners, gloves, bags, hats and accessories) was catalogued to determine potential local sources of synthetic particles into the Ross Island region (Table A1).
2.6 Trajectory analysis
Because samples were collected during or shortly after a single snowfall event (Fig. 2), air mass trajectories were produced to understand potential source regions. Lagrangian air parcel trajectories were derived using the Hybrid Single Particle Lagrangian Integrated Trajectory Model (HYSPLIT) (Stein et al., 2015). HYSPLIT was run using meteorological data from the National Oceanic and Atmospheric Administration Global Forecast System (GFS), with a horizontal grid resolution of 0.25∘ × 0.25∘ and a temporal resolution of 3 h. To achieve this temporal resolution the GFS model is initialized from observational data every 6 h, and then the 3 h forecast is included in the GFS output to fill the gaps in time (Stein et al., 2015).
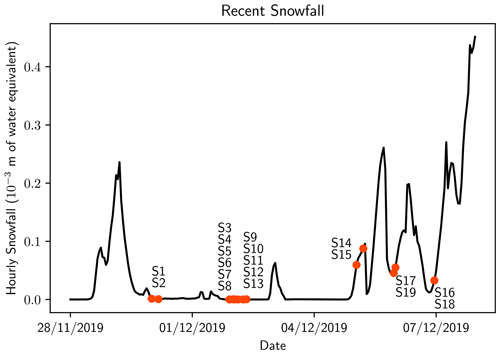
Figure 2Snowfall over the measurement period from the ERA5 reanalysis. These values are averaged over the sample site area (77.75–78∘ S, 166.5–167.25∘ E). Orange circles indicate the time when samples were taken with nearby text indicating the sample number.
The ensemble configuration of HYSPLIT was used to generate 27-member back-trajectory ensembles which represent the uncertainty in the trajectories. For each ensemble trajectory, the meteorological data were offset in the x and/or y directions by one grid point and/or 0.1σ units in the z direction, with 27 ensemble members covering all possible combinations of these offsets. Back trajectories were run from starting points at each of the sampling sites at the corresponding time of sampling and with starting heights of 500, 1000 and 2000 m. Recent published results show that the residence time for microplastic particles in the atmosphere may vary between 1 and 156 h (Brahney et al., 2021). As such, the back trajectories were run for 156 h to show the full range of possible sources.
3.1 Blanks and sample extractions
Recovery rates for spiked samples were 100 %. Across the sample controls an average of 1.5 ± 0.89 particles were found in daily laboratory blanks (n=11), 3 ± 0 in field blanks (n=2) and 2 ± 1 particles in method controls (n=2). All fragments identified in field and laboratory blanks matched the colour and coating of the sampling bottles. No fragments were found in the daily blanks, meaning fibres were the predominant morphotype (Table S1). Spectroscopic analysis confirmed that the outside bottle coating and fragments found in the blanks were polymethyl methacrylate (PMMA). Suspected microplastic particles in field samples of an identical colour and morphotype to those detected in the field and laboratory blanks were not analysed further and were discounted from the results. All reported PMMA still included in results was a different morphotype and colour to the corresponding sampling bottles, and therefore some PMMA is still shown in results. The lining of the lids of the sampling bottles was confirmed as clear silicone, which was not found in any of the samples or blanks. Particles with identical characteristics to those found in the field and laboratory blanks were excluded from further study, and daily blank contamination was subtracted from the results of corresponding samples.
3.2 Concentration of microplastics in snow samples
Microplastics were found in all Antarctic snow samples with a total of 109 particles confirmed as microplastics using µFTIR spectroscopy across the 19 field samples (Fig. 1). Microplastics were present at an average concentration of 29.4 ± 4.7 particles L−1 of melted snow (mean ± 1 standard error) across all sites (Fig. 3). The average microplastic concentration was 22.5 ± 4.0 and 47.2 ± 8.4 particles L−1 at remote sites and base sites, respectively (Fig. 3, Supplement Fig. S1). The highest concentration found was 82 particles L−1 at site S16 (Scott Base), and the lowest concentration found was 4 particles L−1 at site S2 (Erebus Glacier Tongue).
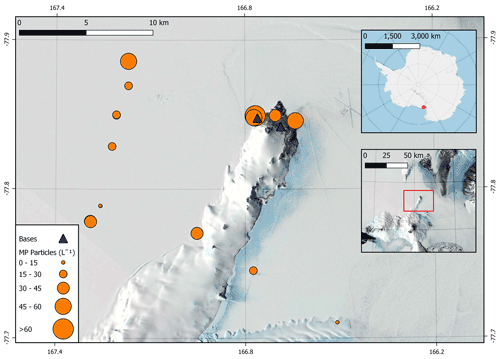
Figure 3Concentrations of microplastics (MP; in particles L−1) at each sampling site in the Ross Island region. Insets provide an overview of the location of sampling sites in Antarctica. Map data sourced from Matsuoka et al. (2021).
3.3 Characterization of microplastics
A total of 13 different polymer types were identified across the snow samples when compared against a spectral reference library (Supplement Fig. S2). Polyethylene terephthalate (PET) was the most frequently detected polymer type, found in 79 % of the samples, comprising 41 % of total polymers identified (Fig. 4a). Copolymers (CP, those containing two or more different monomers) equated to 17 % of total polymers identified (Fig. 4a and Table ). Polymer types comprising less than 10 % of the total polymers identified included polymethyl methacrylate (PMMA, 9 %), polyvinyl chloride (PVC, 9 %), polyamide (PA, 6 %), polyethylene (PE, 4 %), alkyd (ALK, 4 %), cellulose nitrate (CN, 4 %) and other (6 %). Polymer types present in the “other” category with a detection frequency less than 4 % include polytetrafluoroethylene (PTFE), polyvinylidene, polypropylene (PP), silicone and polymethyl anhydride. The cellulose nitrate particles detected were not found in laboratory control samples and did not match the morphotype and colour of the filters used in the digestion stage and so were included in results.
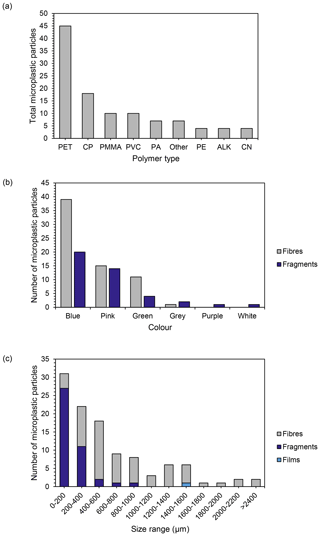
Figure 4Polymer types identified across all samples. (a) Number of each polymer type found across all Antarctic study sites (PET: polyethylene terephthalate; CP: copolymers; PMMA: polymethyl methacrylate; PVC: polyvinyl chloride; PA: polyamide; other: polytetrafluoroethylene (PTFE), polyvinylidene, polypropylene, silicone and polymethyl anhydride; PE: polyethylene; ALK: alkyds; CN: cellulose nitrate); (b) number of microplastic fragments and fibres identified in each colour category (films (n=1) excluded); (c) size distribution of microplastics across all samples categorized by morphotype (length).
Particles confirmed spectroscopically as microplastics were classified as fibres, fragments and films (Supplement Fig. S3). No beads were detected. Fibres were the most abundant morphotype (61 %, Fig. 4), and the majority of microplastics identified were blue (55 %) and pink (23 %, Fig. 4b). Blue was the most common colour of both fibres and fragments. The size range of microplastics detected varied between 50 and 3510 µm with an overall average size of 606 µm (Fig. 4). A large proportion of microplastics were < 1000 µm (81 %), with 28 % in the 0–200 µm size range (Fig. 4). All fragments were ≤ 1000 µm, while fibres were present in all size groups (Fig. 4). The mean size for fragments was 200 µm, while the mean size for fibres was 850 µm, which skews the total averages (Fig. 4).
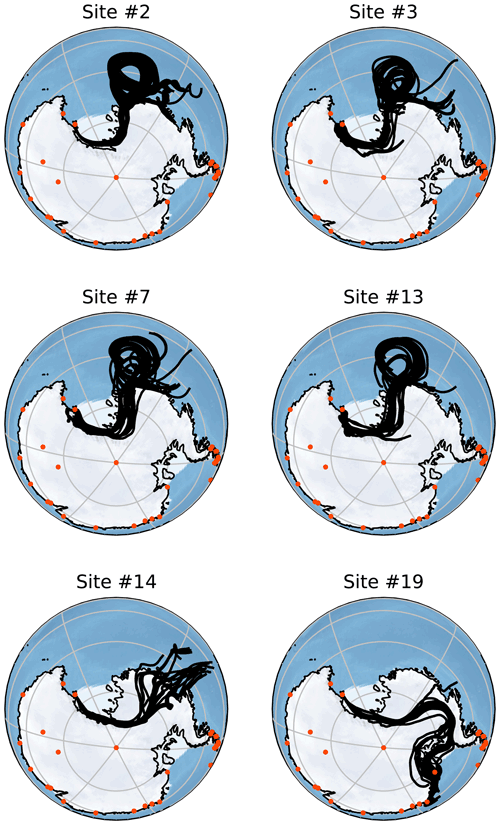
Figure 5Back-trajectory ensembles generated by HYSPLIT for six of the collected samples. Each of these trajectories is run for 156 h with the ensemble members generated by minor perturbations as described in Sect. 2.6. Orange points indicate the locations of active Antarctic research stations that operate all year. All of these trajectories are started at 2000 m altitude but show very similar results to those started at 500 and 1000 m. Note that each simulation is run to correspond to the time of sampling and so is host to different meteorological conditions than other simulations.
3.4 Snowfall and back trajectories
All of the samples from remote sites (S1–S13) were collected over 3 d (30 November–2 December 2019). A large snowfall event occurred 1 d prior to sampling on 30 November 2019 (Fig. 2), and no snowfall was reported over the subsequent 2 d; therefore all remote samples originated from the same snowfall event. Because only the top 2 cm of snow was collected, the microplastics we found were likely deposited in the recent snowfall event.
Figure 5 shows Lagrangian back trajectories beginning at the time and location of six of the different sampling sites. The six events shown were chosen to cover the largest spatial distances and time periods within the dataset, with many of the excluded sites showing near-identical results to included sites. This figure only shows the results for trajectories that start with an altitude of 2000 m, but trajectories initialized at 500 and 1000 m show very similar results (Figs. A1 and A2). Distances travelled by air masses over 6, 24 and 156 h are shown in Table A3 to represent a range of potential airborne microplastic residence times. The residence time is the length of time that a particle can remain in the atmosphere which was estimated by Brahney et al. (2021) to be as long as 156 h for microplastics prior to deposition at the sampling site. We acknowledge that microplastics could be suspended in air for longer than the time periods used, although unlikely, and that they could have been derived from further afield or have been deposited and resuspended from land or the sea en route.
The trajectories presented in Fig. 5 indicate that the snowfall event was likely linked to a Ross air stream event, in which strong near-surface winds flow from the southeast parallel to the Trans-Antarctic Mountains (Parish et al., 2006). The Ross air stream is primarily fed by air from the Siple Coast region in West Antarctica, caused by a complex mixture of katabatic winds and barrier flows along the Trans-Antarctic Mountains created by cyclones (Seefeldt and Cassano, 2012). Pressure gradients induced by cyclones to the north of the ice shelf force air to flow towards the Trans-Antarctic Mountains, but the resulting wind lacks the kinetic energy to pass over the mountains causing a barrier flow. These conditions occur approximately 24 % of the time, though they are more common in the Austral winter, and bring warmer temperatures and strong winds to the Ross Island region (Coggins et al., 2014) along with clouds connected to snowfall (Jolly et al., 2018). The splitting of the Ross air stream around Ross Island also impacts the formation of the Ross Sea polynya and McMurdo Sound polynya (Dale et al., 2017; Brett et al., 2020). These open-water regions provide a possible secondary local source of microplastics; in particular, the McMurdo Sound polynya is very close to site S2. The back trajectories shown in Fig. A1 for Sites 3, 7 and 13 could mean that the open water in the Ross Sea polynya is a relevant source.
In accordance with the climatology of the region, all of the trajectories show that the immediate source of the airflow is from the south following the Trans-Antarctic Mountains. This means that for the majority of these samples, short-term local transport is the most likely source of microplastics as the sampling sites are mostly north of the local bases (Scott Base and McMurdo Base). It is also possible that local small-scale transport processes that are not captured by HYSPLIT could play a key role in transport at this scale. For the trajectories that clearly show a Ross air stream event (sites 2, 3, 7 and 13), the most likely distant sources are the Ross and Amundsen seas as there are no manned stations or other likely sources along the trajectory path. The transport processes in this region can be very rapid with the trajectories from these sites on average covering a distance of 143 km in the first 6 h and 469 km in 24 h. With a 156 h residence time, transport over thousands of kilometres is possible.
For site 19 there is a different set of meteorological conditions causing a divergence from the results of the other samples. The corresponding trajectories show a wide range of possible sources including the Antarctic Peninsula and Weddell Sea. While these conditions are relatively rare compared with the kind of Ross air stream flow examined earlier, they still provide a possible source of microplastics. These different conditions expand the set of possible sources to include more distant sources including many other Antarctic research stations. Given that an assumed maximum residence time of 156 h leads to trajectories covering a total distance of over ∼ 4800 km in transit (Table A3), transport from these stations appears possible but unlikely.
4.1 Microplastic concentrations
Our work provides the first evidence of microplastics in Antarctic snow, and critically, the average concentration of microplastics found in this study are higher (29.4 ± 4.7 particles L−1) than in the surrounding Ross Sea (1.7 × 10−4 particles L−1) and those reported in East Antarctic sea ice (11.7 particles L−1) (Kelly et al., 2020; Cincinelli et al., 2017). This dataset is limited by the low sample volumes due to the permitting restrictions for Antarctic samples. Therefore, we recommend this study be replicated to further understand these preliminary findings. Larger volumes of snow (≥ 10 L) or replicates from the same study sites would be beneficial for future research. Our reported concentrations of microplastics in surface snow near two Antarctic stations (47.2 ± 8.4 particles L−1; highest concentration 82.1 particles L−1) identified similar concentrations to those present in supraglacial debris from an Italian glacier (74 particles kg−1 dry weight) (Ambrosini et al., 2019). For comparison, concentrations of microplastics in Arctic sea ice have been shown to range from 8 to 41 particles L−1 (Geilfus et al., 2019), while Arctic snow sampling identified higher concentrations of microplastics of up to 14.4 × 103 particles L−1 (Bergmann et al., 2019), which may be attributed to the proximity to more populated regions.
4.2 Microplastic characteristics
The most frequent polymer type we detected was PET, which was found in 79 % of all samples. Approximately 60 % of all PET produced is used for synthetic fibres and 30 % for plastic bottles (Ji, 2013). Our results differ to surrounding marine studies which found PE and PP were the most common polymer types in seawater samples from the Ross Sea region (Cincinelli et al., 2017). Comparatively, varnish (including acrylates) and rubber were the most common in Arctic snow collected using similar sampling techniques, with PMMA being the third most common polymer type in this study (Bergmann et al., 2019). Air samples collected over the West Pacific Ocean similarly found PET was present in the highest abundance (57 %) (Liu et al., 2019b).
Fibres were the most abundant morphotype (60 %) followed by fragments (39 %) and films (1 %, Fig. 4). These findings are consistent with previous studies whereby fibres were also the dominant morphotype of airborne microplastics due to their relatively low density and physical characteristics (Liu et al., 2019a; Dris et al., 2016; Bullard et al., 2021). Analysis of seawater samples in the surrounding Ross Sea identified fragments as the predominant morphotype (72 %) (Cincinelli et al., 2017), in contrast to our results. Fibres were the most predominant morphotype in sediment from Terra Nova Bay (Munari et al., 2017), which is consistent with the findings of this research. This likely indicates that the distribution of microplastics around the Ross Sea region is heterogeneous depending on the sample type. Further research is required to understand the microplastic footprint in the region. Similar to previous studies, we identified darker colours (blue, black and navy) as the most common (Ambrosini et al., 2019; Liu et al., 2019a, b). Dark-coloured microplastics are likely efficient at absorbing solar radiation compared to lighter colours and are of particular concern in the cryosphere as they may accelerate melting (Evangeliou et al., 2020).
As the size distribution of identified microplastics is skewed towards smaller particles (Fig. 4), it is likely that particles smaller than the smallest particle observed (50 µm) are present but not able to be detected due to the magnification limit of the stereomicroscope (20 µm) and difficulties in handling particles < 50 µm. The abundance of microplastics has previously been shown to increase with decreasing size (Isobe et al., 2017; Levermore et al., 2020), which corresponds to the findings of this study (Fig. 4). The size distribution from our study was comparable to those measured in the remote Pyrenees (Allen et al., 2019). We identified that only 10 % of the microplastics were < 100 µm, while findings in the Arctic reported 98 % of particles were < 100 µm (Bergmann et al., 2019). While fibres were present across all size ranges found, the only fragments found were smaller than 1000 µm, consistent with previous studies (Revell et al., 2021 and references therein).
4.3 Origin of microplastics
Microplastics in Antarctica may originate from both local sources and long-range transport. Direct sources of microplastics to the Antarctic environment may include fragmentation of plastic equipment from research stations, clothing worn by base staff and researchers, and mismanaged waste. Microplastics may also enter the Antarctic environment via long-range transport by ocean currents (Fraser et al., 2018), ocean to atmosphere exchange (Allen et al., 2020), and both short- and long-range atmospheric transportation (Evangeliou et al., 2020; Brahney et al., 2021).
4.3.1 Local sources of microplastics
Antarctic research stations on Ross Island, Scott Base (NZ) and McMurdo Station (US) have the closest proximity to the sampling sites, up to 20 km away (Fig. 1), with Zucchelli Station (Italian) the next closest at 350 km away, which is only operational over summer. Direct transport from Zucchelli Station is highly unlikely given that it is north from our sampling sites, and the winds primarily come from the south. McMurdo Station has a maximum capacity of 1200 people in the summer months and decreases to approximately 150 people over winter, whereas Scott Base has a capacity of 86 people over summer and typically hosts 11 staff over winter (COMNAP, 2017). The concentration of microplastics measured at the base sites (S14–S19; 47 ± 8 particles L−1) was higher on average than the remote sites (22 ± 4 particles L−1), suggesting that microplastics originated from local sources.
Plastic products in use at research stations (including building materials, marker flags, safety equipment and tyre rubber) may fragment with environmental exposure and be a potential local source of microplastics into the environment. General wear and weathering from clothing and outdoor equipment used in the field may introduce plastics into more remote regions away from populated bases. In addition, enhanced ultraviolet fluxes due to the Antarctic ozone hole may accelerate the fragmentation of larger plastic products into microplastics (Williamson et al., 2019).
PET was the most common polymer found in snow samples making up 41 % of total microplastics. The most common polymer found in catalogued gear was also PET, which was present in 48 % of the garments in varying percentages from 68 % to 100 %, followed by PA which was present in 30 % of the garments (Table A1). Fibres were also the most common morphotype in snow samples (Fig. 4), suggesting an origin from textile garments (Acharya et al., 2021). The most common colours used in the clothing are black, orange, navy and grey (Table ). These colours are consistent with those found in snow samples, with 55 % of the particles being of a dark colour (black, blue, navy; Fig. 4b). Throughout some regions of Antarctica, polyamide flags are used to identify safe routes for travel and are visible in harsh weather conditions. Weathering of wayfinding flags may release microplastics into the environment, and due to the high volume used each year, it could have the potential to impact the surrounding environment. The flags are predominantly red, green, blue and black, with weathering processes resulting in a dulling of the flag colour to a pale pink or blue (Fig. 6). In this study, blue was the most common colour detected (55 %), followed by pink (23 %, Fig. 4b). Replacing the polyamide fabric used for wayfinding flags with an alternative non-synthetic material could reduce the local impact of microplastics on the environment caused by research stations in Antarctica.
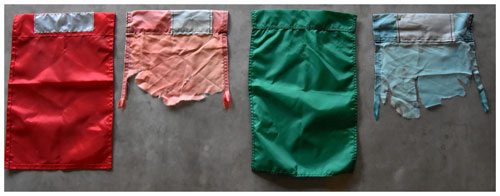
Figure 6Marker flags used in Antarctica. Before being placed outside (left) and after they were retrieved (right). Photo credit: Evan Townsend (https://www.truesouthflag.com/flags-of-antarctica, last access: 25 May 2022).
Wastewater treatment plants (WWTPs) have been identified as a source of entry of microplastics to the environment worldwide (Browne et al., 2011). An excess of 700 000 synthetic fibres are released from an average 6 kg acrylic fibre load of washing (Napper and Thompson, 2016), and with tumble dryers being present these may also contribute to the presence of microplastics (Tao et al., 2022). The WWTP at Zucchelli Station has been hypothesized as a potential source of microplastic fibres into the Ross Sea when identifying microplastics in nearby benthic sediment (Cincinelli et al., 2017); however, no WWTPs in Antarctica have been investigated for their contribution of microplastics to the environment to date. The Protocol on Environmental Protection to the Antarctic Treaty (Annex III and Annex IV) entered into force in 1998. The protocol prohibits plastic waste from being released by ocean vessels within the Antarctic Treaty area and untreated sewage within 12 nautical miles of land or ice shelves. However, no annex for the release of effluent from research stations was established. WWTPs are not mandatory at stations in Antarctica, leaving many research stations with insufficient wastewater treatment facilities (Gröndahl et al., 2009; Stark et al., 2016). The findings of the Antarctic Treaty Consultative Meeting (ATCM) in 2019 recommended that all governments with Antarctic interests eliminate products containing microplastic beads, work towards reducing microplastic release from wastewater systems and support monitoring of plastic pollution regarding human activity. Future work should focus on quantifying the contribution of tumble dryers and wastewater discharge to the abundance of microplastics in Antarctica, as well as the most effective wastewater treatment process(es) for microplastic removal, which could ultimately be used at these bases.
4.3.2 Long-range transport of microplastics
The transportation of particulates, such as dust, across the Southern Ocean and into Antarctica from other continents has been explored in previous literature, identifying mid-latitude circumpolar westerly winds dominating atmospheric transport to these regions, with Patagonia and New Zealand the most likely sources (Neff and Bertler, 2015). Transportation of microplastics into the Southern Ocean via deep water processes can also introduce synthetic pollutants from afar (Mountford and Maqueda, 2020). Atmospheric long-range transport has been identified as a contamination source of persistent organic pollutants into Antarctica (Kallenborn et al., 2013), as well as dust originating from Australia, Patagonia and the Northern Hemisphere (Li et al., 2008).
Short-range transport of microplastics from the bases to sampling sites close by (e.g. S14–S19) is more likely than long-range transport, given the sites' proximity to research bases and the climatology of the area. Yet sites further away may have more influence from long-range transportation, showing the potential influence of both short-range and long-range inputs. HYSPLIT trajectory modelling indicates that microplastics may have travelled from the Amundsen or Ross seas to reach the remote sample sites and could possibly have come from as far as the Weddell Sea (based on hypothesized particle residence time in Brahney et al., 2021). There are no significant anthropogenic sources identified along the trajectories of the majority of the air masses, such as other research stations, with the Ross Ice Shelf extending for approximately 800 km westward from the sampling sites.
Microplastic particles may have originated from local anthropogenic sources and been transported around Antarctica via a cycle of entrainment and deposition. Alternatively, microplastics may have originated from surface waters surrounding Antarctica via co-emission with sea spray (Allen et al., 2020) and atmospheric transport. Given that the air mass trajectories pass through the Ross Sea, Amundsen Sea and potentially the Weddell Sea, these are all possible sources with the known presence of plastics and microplastics at these locations (Cincinelli et al., 2017; Munari et al., 2017; Van Cauwenberghe et al., 2013; Barnes et al., 2010). Recent atmospheric transport modelling indicates that Antarctica is a net importer of microplastics, with the flux of microplastics from mismanaged plastic waste in the ocean transferring to the atmosphere at the Antarctic coast likely exceeding anthropogenic sources of microplastics on the continent (Brahney et al., 2021).
4.4 Implications and outlook
The implications of microplastics reaching remote regions such as Antarctica are vast. Antarctic organisms have adapted to extreme environmental conditions over many millions of years (Peck, 2018), and the rapid environmental changes due to anthropogenic influence are threatening the unique ecosystems present in the polar regions (Aronson et al., 2011; Convey and Peck, 2019; Lee et al., 2017). Organisms' exposure to microplastics can lead to limited growth, negative effects on reproduction and impaired biological functions (Foley et al., 2018), increasing pressure on ecosystems. Epiplastic communities of bacteria, microalgae and invertebrates can form on environmental plastic particles, which may contribute to the movement and introduction of invasive species into the Antarctic region (Lacerda et al., 2019, 2020).
Negative effects of plastic pollution in Antarctic waters have been reported since 1990 when anthropogenic products, mainly from fishing vessels, were found to be entangling fur seals (Croxall et al., 1990). The ingestion of microplastics by zooplankton, an ecologically important organism in marine ecosystems, can disrupt usual biological processes and negatively impact upon function and health (Cole et al., 2013). The ingestion of microplastics by Antarctic krill may cause a multitude of negative effects on the entire Antarctic food chain as they are a keystone species which a large number of organisms rely on to survive in the Southern Ocean (Hill et al., 2006). Plastic ingestion by the common Antarctic collembolan (Cryptopygus antarcticus) has also been identified, indicating the presence of plastic in the Antarctic terrestrial food chain (Bergami et al., 2020).
The consumption of microplastics by higher predators in the Antarctic ecosystem has also been noted, with gentoo (Pygoscelis papua), Adélie (Pygoscelis adeliae), chinstrap (Pygoscelis antarcticus) and king (Aptenodytes patagonicus) penguins showing the presence of microplastics in their diet (Fragão et al., 2021; Le Guen et al., 2020). The atmospheric transport of microplastics increases their availability to terrestrial organisms with the potential for ingestion and consequently negative health effects. Endemic Antarctic penguin species could be exposed to microplastics during their breeding season which is spent on Antarctic fast ice with their colonies. The compounding effects of anthropogenic changes are putting Emperor penguin species at risk with current models predicting a population decline of 81 % by 2100 (Jenouvrier et al., 2019).
Microplastics are an emerging contaminant for which appropriate controls and regulations have not been widely put in place (Waller et al., 2017). Members of the Antarctic Treaty committed to reducing plastic pollution in Antarctica and the Southern Ocean at the Antarctic Treaty Consultative Meeting in 2019, urging Antarctic activities to reduce their use of plastic care products containing microplastics, reduce release of microplastics from WWTP and support greater monitoring of plastic pollution in the region. As microplastics continue to pose a growing threat to the Antarctic ecosystem, more rapid approaches are required throughout the Antarctic Treaty System to minimize the widespread impacts. Further research is required to determine the inputs of microplastics into the Antarctic environment, as well as developing a greater understanding of the role of long-range transport for microplastic distribution.
The findings of this study highlight the global reach of plastic pollution and identifies the need for urgency in creating successful policy to reduce its extent and effects, both globally and locally. While studies in the Antarctic region currently focus on marine microplastic pollution, future research and policy need to take a holistic approach to incorporate airborne and terrestrial impacts. The Protocol on Environmental Protection to the Antarctic Treaty (1991) aims to promote the protection of the Antarctic environment and its place in the world as a natural reserve devoted to peace and science. Growing rates of pollution across the world make this a much greater challenge, and huge transdisciplinary efforts are required to ensure this can continue to be achieved.
This study confirms the presence of microplastics in Antarctic snow. Microplastics were identified on the Ross Ice Shelf and near Scott Base and McMurdo Station at an average concentration of 29 particles L−1. Fibres were the most common morphotype identified, and PET was the most common polymer found. Outdoor clothing and other equipment used at the nearby research stations was catalogued to understand local inputs of microplastics while also assessing the potential for long-range transport through back-trajectory modelling. Back trajectories indicated that microplastics may have travelled a distance of up to 6000 km, depending on the residence time. Given that air masses passed over the bases prior to sampling and that the polymers we identified are consistent with those catalogued, it is likely that local inputs were a contributor to the microplastic pollution identified. Our results highlight the importance of further monitoring – both in Antarctica and in surrounding waters – to develop our understanding of the microplastic footprint in Antarctica and the threat it may pose to the Antarctic environment.
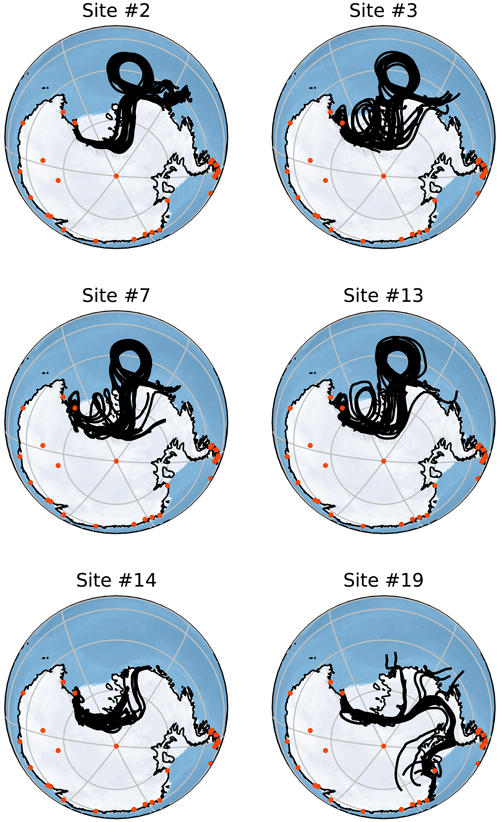
Figure A1Like Fig. 5 but trajectories were initialized with a starting height of 500 m.
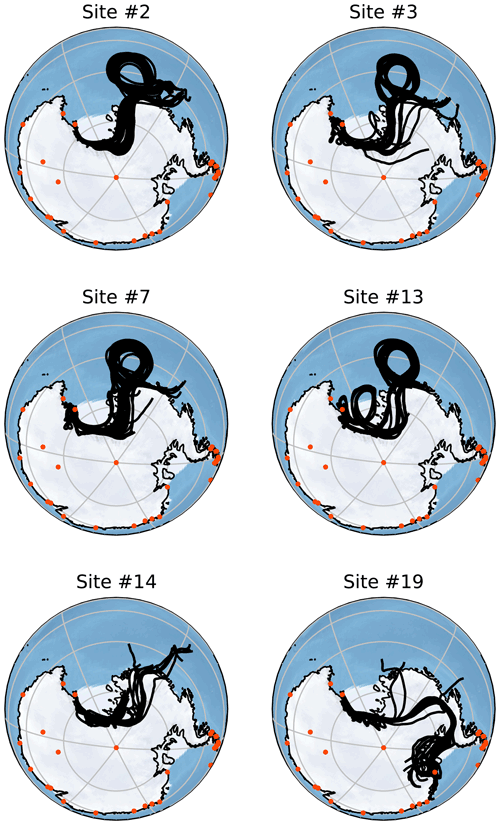
Figure A2Like Fig. 5 but trajectories were initialized with a starting height of 1000 m.
Table A2Characteristics of microplastics identified and sample volume. As discussed in the text, “blue” includes blue, black and navy. “Size” indicates the width for fragments and length for fibres.
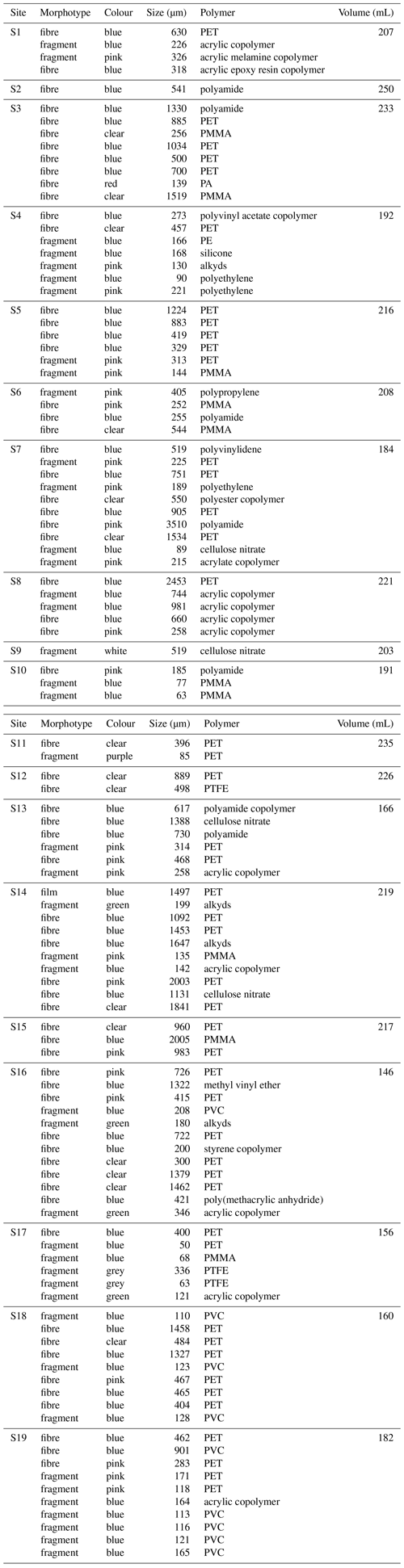
The microplastic data generated in this study are provided in the Appendix of this paper, including microplastic counts, sample volume, particle size, shape and polymer type. Relevant data to evaluate the conclusions of this paper are present in either the main paper, the Appendix or the Supplement.
The supplement related to this article is available online at: https://doi.org/10.5194/tc-16-2127-2022-supplement.
Conceptualization of the study was completed by LER and SG. Methodology development was undertaken by LER, HR and SG. Field sampling was completed by ARA, ML and AJM. Investigation was undertaken by ARA, NEW and AS. Visualization was completed by ARA, NEW, ML and AS. Supervision of study was led by LER and SG. The original draft was completed by ARA and LER with review and editing completed by all authors.
The contact author has declared that neither they nor their co-authors have any competing interests.
Publisher’s note: Copernicus Publications remains neutral with regard to jurisdictional claims in published maps and institutional affiliations.
The authors thank Nicole Lauren-Manuera, Alex Nicholls, Paula Brooksby, Justin Harrison, Antarctica New Zealand, staff and students from Gateway Antarctica, and the Postgraduate Certificate of Antarctic Studies 2019 group. Alex R. Aves was supported by Gateway Antarctica's Ministry of Foreign Affairs and Trade Scholarship in Antarctic and Southern Ocean Studies. We acknowledge mana whenua, Ngāi Tūāhuriri, on whose lands our analysis and writing took place.
This research has been supported by the Marsden Fund (grant no. MFP-UOC1903).
This paper was edited by Kaitlin Keegan and reviewed by two anonymous referees.
Absher, T. M., Ferreira, S. L., Kern, Y., Ferreira, A. L., Christo, S. W., and Ando, R. A.: Incidence and identification of microfibers in ocean waters in Admiralty Bay, Antarctica, Environ. Sci. Pollut. Res., 26, 292–298, 2019. a
Acharya, S., Rumi, S. S., Hu, Y., and Abidi, N.: Microfibers from synthetic textiles as a major source of microplastics in the environment: A review, Text. Res. J., 91, 2136–2156, 2021. a
Allen, S., Allen, D., Phoenix, V. R., Roux, G. L., Jiménez, P. D., Simonneau, A., Binet, S., and Galop, D.: Atmospheric transport and deposition of microplastics in a remote mountain catchment, Nat. Geosci., 12, 339–344, https://doi.org/10.1038/s41561-019-0335-5, 2019. a, b, c
Allen, S., Allen, D., Moss, K., Le Roux, G., Phoenix, V. R., and Sonke, J. E.: Examination of the ocean as a source for atmospheric microplastics, PloS one, 15, e0232746, https://doi.org/10.1371/journal.pone.02327, 2020. a, b
Ambrosini, R., Azzoni, R. S., Pittino, F., Diolaiuti, G., Franzetti, A., and Parolini, M.: First evidence of microplastic contamination in the supraglacial debris of an alpine glacier, Environ. Pollut., 253, 297–301, 2019. a, b
Aronson, R. B., Thatje, S., McClintock, J. B., and Hughes, K. A.: Anthropogenic impacts on marine ecosystems in Antarctica, Ann. NY Acad. Sci., 1223, 82–107, 2011. a
Barnes, D. K., Walters, A., and Gonçalves, L.: Macroplastics at sea around Antarctica, Mar. Environ. Res., 70, 250–252, 2010. a
Bergami, E., Rota, E., Caruso, T., Birarda, G., Vaccari, L., and Corsi, I.: Plastics everywhere: first evidence of polystyrene fragments inside the common Antarctic collembolan Cryptopygus antarcticus, Biol. Lett., 16, 20200093, https://doi.org/10.1098/rsbl.2020.0093, 2020. a
Bergmann, M., Mützel, S., Primpke, S., Tekman, M. B., Trachsel, J., and Gerdts, G.: White and wonderful? Microplastics prevail in snow from the Alps to the Arctic, Sci. Adv., 5, eaax1157, https://doi.org/10.1126/sciadv.aax1157, 2019. a, b, c, d, e, f
Brahney, J., Hallerud, M., Heim, E., Hahnenberger, M., and Sukumaran, S.: Plastic rain in protected areas of the United States, Science, 368, 1257–1260, https://doi.org/10.1126/science.aaz5819, 2020. a, b
Brahney, J., Mahowald, N., Prank, M., Cornwell, G., Klimont, Z., Matsui, H., and Prather, K. A.: Constraining the atmospheric limb of the plastic cycle, P. Natl. Acad. Sci. USA, 118, e2020719118, https://doi.org/10.1073/pnas.2020719118, 2021. a, b, c, d, e, f
Brander, S. M., Renick, V. C., Foley, M. M., Steele, C., Woo, M., Lusher, A., Carr, S., Helm, P., Box, C., and Cherniak, S.: Sampling and quality assurance and quality control: a guide for scientists investigating the occurrence of microplastics across matrices, Appl. Spectrosc., 74, 1099–1125, 2020. a
Brett, G., Irvin, A., Rack, W., Haas, C., Langhorne, P., and Leonard, G.: Variability in the distribution of fast ice and the sub-ice platelet layer near McMurdo Ice Shelf, J. Geophys. Res.-Ocean., 125, e2019JC015678, https://doi.org/10.1029/2019JC015678, 2020. a
Bridson, J. H., Patel, M., Lewis, A., Gaw, S., and Parker, K.: Microplastic contamination in Auckland (New Zealand) beach sediments, Mar. Pollut. Bull., 151, 110867, https://doi.org/10.1016/j.marpolbul.2019.110867, 2020. a
Browne, M. A., Crump, P., Niven, S. J., Teuten, E., Tonkin, A., Galloway, T., and Thompson, R.: Accumulation of microplastic on shorelines worldwide: sources and sinks, Environ. Sci. Technol., 45, 9175–9179, 2011. a
Bullard, J. E., Ockelford, A., O'Brien, P., and Neuman, C. M.: Preferential transport of microplastics by wind, Atmos. Environ., 245, 118038, https://doi.org/10.1016/j.atmosenv.2020.118038, 2021. a
Cai, L., Wang, J., Peng, J., Tan, Z., Zhan, Z., Tan, X., and Chen, Q.: Characteristic of microplastics in the atmospheric fallout from Dongguan city, China: preliminary research and first evidence, Environ. Sci. Pollut. Res., 24, 24928–24935, https://doi.org/10.1007/s11356-017-0116-x, 2017. a
Cincinelli, A., Scopetani, C., Chelazzi, D., Lombardini, E., Martellini, T., Katsoyiannis, A., Fossi, M. C., and Corsolini, S.: Microplastic in the surface waters of the Ross Sea (Antarctica): occurrence, distribution and characterization by FTIR, Chemosphere, 175, 391–400, 2017. a, b, c, d, e, f
Coggins, J. H., McDonald, A. J., and Jolly, B.: Synoptic climatology of the Ross Ice Shelf and Ross Sea region of Antarctica: k‐means clustering and validation, Int. J. Climatol., 34, 2330–2348, 2014. a
Cole, M., Lindeque, P., Fileman, E., Halsband, C., Goodhead, R., Moger, J., and Galloway, T. S.: Microplastic ingestion by zooplankton, Environ. Sci. Technol., 47, 6646–6655, 2013. a
COMNAP: Antarctic Station Catalogue, COMNAP, https://static1.squarespace.com/static/ (last access: 20 March 2022), 2017. a
Convey, P. and Peck, L. S.: Antarctic environmental change and biological responses, Sci. Adv., 5, eaaz0888, https://doi.org/10.1126/sciadv.aaz0888, 2019. a
Cózar, A., Echevarría, F., González-Gordillo, J. I., Irigoien, X., Úbeda, B., Hernández-León, S., Palma, Á. T., Navarro, S., García-de Lomas, J., Ruiz, A., Fernández-de Puelles, M. L., and Duarte, C. M.: Plastic debris in the open ocean, P. Natl. Acad. Sci. USA, 111, 10239–10244, 2014. a
Croxall, J., Rodwell, S., and Boyd, I.: Entanglement in man‐made debris of Antarctic fur seals at Bird Island, South Georgia, Mar. Mammal Sci., 6, 221–233, 1990. a
Dale, E. R., McDonald, A. J., Coggins, J. H. J., and Rack, W.: Atmospheric forcing of sea ice anomalies in the Ross Sea polynya region, The Cryosphere, 11, 267–280, https://doi.org/10.5194/tc-11-267-2017, 2017. a
De Frond, H., Rubinovitz, R., and Rochman, C. M.: µATR-FTIR Spectral Libraries of Plastic Particles (FLOPP and FLOPP-e) for the Analysis of Microplastics, Anal. Chem., 93, 15878–15885, 2021. a
de Souza Machado, A. A., Kloas, W., Zarfl, C., Hempel, S., and Rillig, M. C.: Microplastics as an emerging threat to terrestrial ecosystems, Glob. Change Biol., 24, 1405–1416, 2018. a
Dris, R., Gasperi, J., Rocher, V., Saad, M., Renault, N., and Tassin, B.: Microplastic contamination in an urban area: a case study in Greater Paris, Environ. Chem., 12, 592–599, https://doi.org/10.1071/EN14167, 2015. a
Dris, R., Gasperi, J., Saad, M., Mirande, C., and Tassin, B.: Synthetic fibers in atmospheric fallout: a source of microplastics in the environment?, Mar. Pollut. Bull., 104, 290–293, 2016. a, b
Evangeliou, N., Grythe, H., Klimont, Z., Heyes, C., Eckhardt, S., Lopez-Aparicio, S., and Stohl, A.: Atmospheric transport is a major pathway of microplastics to remote regions, Nat. Commun., 11, 1–11, 2020. a, b, c, d
Foley, C. J., Feiner, Z. S., Malinich, T. D., and Höök, T. O.: A meta-analysis of the effects of exposure to microplastics on fish and aquatic invertebrates, Sci. Total Environ., 631, 550–559, 2018. a
Fragão, J., Bessa, F., Otero, V., Barbosa, A., Sobral, P., Waluda, C. M., Guímaro, H. R., and Xavier, J. C.: Microplastics and other anthropogenic particles in Antarctica: using penguins as biological samplers, Sci. Total Environ., 788, 147698, https://doi.org/10.1016/j.scitotenv.2021.147698, 2021. a
Fraser, C. I., Morrison, A. K., Hogg, A. M., Macaya, E. C., van Sebille, E., Ryan, P. G., Padovan, A., Jack, C., Valdivia, N., and Waters, J. M.: Antarctica’s ecological isolation will be broken by storm-driven dispersal and warming, Nat. Clim. Change, 8, 704–708, 2018. a
Ganguly, M. and Ariya, P. A.: Ice Nucleation of Model Nanoplastics and Microplastics: A Novel Synthetic Protocol and the Influence of Particle Capping at Diverse Atmospheric Environments, ACS Earth Space Chem., 3, 1729–1739, 2019. a
Geilfus, N.-X., Munson, K., Sousa, J., Germanov, Y., Bhugaloo, S., Babb, D., and Wang, F.: Distribution and impacts of microplastic incorporation within sea ice, Mar. Pollut. Bull., 145, 463–473, 2019. a
González-Pleiter, M., Edo, C., Velázquez, D., Casero-Chamorro, M. C., Leganés, F., Quesada, A., Fernández-Piñas, F., and Rosal, R.: First detection of microplastics in the freshwater of an Antarctic Specially Protected Area, Mar. Pollut. Bull., 161, 111811, https://doi.org/10.1016/j.marpolbul.2020.111811, 2020. a
Gordon, A. L.: Antarctic polar front zone, Antarctic Oceanology I, Antarctic Res. Ser., 15, 205–221, 1971. a
Gröndahl, F., Sidenmark, J., and Thomsen, A.: Survey of waste water disposal practices at Antarctic research stations, Polar Res., 28, 298–306, 2009. a
Hermabessiere, L., Dehaut, A., Paul-Pont, I., Lacroix, C., Jezequel, R., Soudant, P., and Duflos, G.: Occurrence and effects of plastic additives on marine environments and organisms: A review, Chemosphere, 182, 781–793, 2017. a
Hill, S., Murphy, E., Reid, K., Trathan, P., and Constable, A.: Modelling Southern Ocean ecosystems: krill, the food-web, and the impacts of harvesting, Biol. Rev., 81, 581–608, 2006. a
Horton, A. A. and Dixon, S. J.: Microplastics: An introduction to environmental transport processes, WIRES Water, 5, e1268, https://doi.org/10.1002/wat2.1268, 2018. a
Huiskes, A., Convey, P., and Bergstrom, D.: Trends in Antarctic Terrestrial and Limnetic Ecosystems: Antarctica as a Global Indicator, in: Trends in Antarctic Terrestrial and Limnetic Ecosystems, edited by: Bergstrom, D. M., Convey, P., and Huiskes, A. H. L., Springer, Dordrecht, https://doi.org/10.1007/1-4020-5277-4_1, 2006. a
Isobe, A., Uchiyama-Matsumoto, K., Uchida, K., and Tokai, T.: Microplastics in the Southern Ocean, Mar. Pollut. Bull., 114, 623–626, 2017. a, b
Jenouvrier, S., Holland, M., Iles, D., Labrousse, S., Landrum, L., Garnier, J., Caswell, H., Weimerskirch, H., LaRue, M., and Ji, R.: The Paris Agreement objectives will likely halt future declines of emperor penguins, Glob. Change Biol., 26, 1170–1184, 2019. a
Ji, L. N.: Study on preparation process and properties of polyethylene terephthalate (PET), Appl. Mech. Mater. Trans. Tech. Publ., 312, 406–410, 2013. a
Jolly, B., Kuma, P., McDonald, A., and Parsons, S.: An analysis of the cloud environment over the Ross Sea and Ross Ice Shelf using CloudSat/CALIPSO satellite observations: the importance of synoptic forcing, Atmos. Chem. Phys., 18, 9723–9739, https://doi.org/10.5194/acp-18-9723-2018, 2018. a
Kallenborn, R., Breivik, K., Eckhardt, S., Lunder, C. R., Manø, S., Schlabach, M., and Stohl, A.: Long-term monitoring of persistent organic pollutants (POPs) at the Norwegian Troll station in Dronning Maud Land, Antarctica, Atmos. Chem. Phys., 13, 6983–6992, https://doi.org/10.5194/acp-13-6983-2013, 2013. a
Kelly, A., Lannuzel, D., Rodemann, T., Meiners, K., and Auman, H.: Microplastic contamination in east Antarctic sea ice, Mar. Pollut. Bull., 154, 111130, https://doi.org/10.1016/j.marpolbul.2020.111130, 2020. a
Klein, M. and Fischer, E. K.: Microplastic abundance in atmospheric deposition within the Metropolitan area of Hamburg, Germany, Sci. Total Environ., 685, 96–103, https://doi.org/10.1016/j.scitotenv.2019.05.405, 2019. a
Knobloch, E., Ruffell, H., Aves, A., Pantos, O., Gaw, S., and Revell, L. E.: Comparison of Deposition Sampling Methods to Collect Airborne Microplastics in Christchurch, New Zealand, Water Air Soil Pollut., 232, 133, https://doi.org/10.1007/s11270-021-05080-9, 2021. a, b
Kroon, F., Motti, C., Talbot, S., Sobral, P., and Puotinen, M.: A workflow for improving estimates of microplastic contamination in marine waters: A case study from North-Western Australia, Environ. Pollut., 238, 26–38, 2018. a
Lacerda, A. L. d. F., Rodrigues, L. d. S., Van Sebille, E., Rodrigues, F. L., Ribeiro, L., Secchi, E. R., Kessler, F., and Proietti, M. C.: Plastics in sea surface waters around the Antarctic Peninsula, Sci. Rep., 9, 1–12, 2019. a, b
Lacerda, A. L. d. F., Proietti, M. C., Secchi, E. R., and Taylor, J. D.: Diverse groups of fungi are associated with plastics in the surface waters of the Western South Atlantic and the Antarctic Peninsula, Mol. Ecol., 29, 1903–1918, https://doi.org/10.1111/mec.15444, 2020. a
Le Guen, C., Suaria, G., Sherley, R. B., Ryan, P. G., Aliani, S., Boehme, L., and Brierley, A. S.: Microplastic study reveals the presence of natural and synthetic fibres in the diet of King Penguins (Aptenodytes patagonicus) foraging from South Georgia, Environ. Int., 134, 105303, https://doi.org/10.1016/j.envint.2019.105303, 2020. a
Lee, J. R., Raymond, B., Bracegirdle, T. J., Chades, I., Fuller, R. A., Shaw, J. D., and Terauds, A.: Climate change drives expansion of Antarctic ice-free habitat, Nature, 547, 49–54, 2017. a
Levermore, J. M., Smith, T. E., Kelly, F. J., and Wright, S. L.: Detection of microplastics in ambient particulate matter using Raman spectral imaging and chemometric analysis, Anal. Chem., 92, 8732–8740, 2020. a
Li, F., Ginoux, P., and Ramaswamy, V.: Distribution, transport, and deposition of mineral dust in the Southern Ocean and Antarctica: Contribution of major sources, J. Geophys. Res.-Atmos., 113, D10207, https://doi.org/10.1029/2007JD009190, 2008. a
Liu, K., Wang, X., Fang, T., Xu, P., Zhu, L., and Li, D.: Source and potential risk assessment of suspended atmospheric microplastics in Shanghai, Sci. Total Environ., 675, 462–471, 2019a. a, b
Liu, K., Wu, T., Wang, X., Song, Z., Zong, C., Wei, N., and Li, D.: Consistent transport of terrestrial microplastics to the ocean through atmosphere, Environ. Sci. Technol., 53, 10612–10619, 2019b. a, b
MacLeod, M., Arp, H. P. H., Tekman, M. B., and Jahnke, A.: The global threat from plastic pollution, Science, 373, 61–65, 2021. a
Masura, J., Baker, J. E., Foster, G. D., Arthur, C., and Herring, C.: Laboratory methods for the analysis of microplastics in the marine environment: recommendations for quantifying synthetic particles in waters and sediments, Siver Spring, MD, NOAA Marine Debris Division, 31 pp., NOAA Technical Memorandum NOS-OR&R-48, https://doi.org/10.25607/OBP-604, 2015. a
Materić, D., Kasper-Giebl, A., Kau, D., Anten, M., Greilinger, M., Ludewig, E., van Sebille, E., Röckmann, T., and Holzinger, R.: Micro- and Nanoplastics in Alpine Snow: A New Method for Chemical Identification and (Semi)Quantification in the Nanogram Range, Environ. Sci. Technol., 54, 2353–2359, https://doi.org/10.1021/acs.est.9b07540, 2020. a
Materić, D., Ludewig, E., Brunner, D., Röckmann, T., and Holzinger, R.: Nanoplastics transport to the remote, high-altitude Alps, Environ. Pollut., 288, 117697, https://doi.org/10.1016/j.envpol.2021.117697, 2021. a
Mato, Y., Isobe, T., Takada, H., Kanehiro, H., Ohtake, C., and Kaminuma, T.: Plastic resin pellets as a transport medium for toxic chemicals in the marine environment, Environ. Sci. Technol., 35, 318–324, 2001. a
Matsuoka, K., Skoglund, A., Roth, G., de Pomereu, J., Griffiths, H., Headland, R., Herried, B., Katsumata, K., Le Brocq, A., Licht, K., Morgan, F., Neff, P. D., Ritz, C., Scheinert, M., Tamura, T., Van de Putte, A., van den Broeke, M., von Deschwanden, A., Deschamps-Berger, C., Van Liefferinge, B., Tronstad, S., and Melvær, Y.: Quantarctica, an integrated mapping environment for Antarctica, the Southern Ocean, and sub-Antarctic islands, Environ. Model. Softw., 140, 105015, https://doi.org/10.1016/j.envsoft.2021.105015, 2021. a, b
McConnell, J. R., Maselli, O. J., Sigl, M., Vallelonga, P., Neumann, T., Anschütz, H., Bales, R. C., Curran, M. A. J., Das, S. B., Edwards, R., Kipfstuhl, S., Layman, L., and Thomas, E. R.: Antarctic-wide array of high-resolution ice core records reveals pervasive lead pollution began in 1889 and persists today, Sci. Rep., 4, 1–5, 2014. a
Mountford, A. S. and Maqueda, M. M.: Modelling the accumulation and transport of microplastics by sea ice, J. Geophys. Res.-Ocean., 126, e2020JC016826, https://doi.org/10.1029/2020JC016826, 2020. a
Munari, C., Infantini, V., Scoponi, M., Rastelli, E., Corinaldesi, C., and Mistri, M.: Microplastics in the sediments of Terra Nova Bay (Ross Sea, Antarctica), Mar. Pollut. Bull., 122, 161–165, 2017. a, b, c
Napper, I. E. and Thompson, R. C.: Release of synthetic microplastic plastic fibres from domestic washing machines: Effects of fabric type and washing conditions, Mar. Pollut. Bull., 112, 39–45, 2016. a
Neff, P. D. and Bertler, N. A.: Trajectory modeling of modern dust transport to the Southern Ocean and Antarctica, J. Geophys. Res.-Atmos., 120, 9303–9322, 2015. a
Parish, T. R., Cassano, J. J., and Seefeldt, M. W.: Characteristics of the Ross Ice Shelf air stream as depicted in Antarctic Mesoscale Prediction System simulations, J. Geophys. Res.-Atmos., 111, D12109, https://doi.org/10.1029/2005JD006185, 2006. a
Peck, L. S.: Antarctic marine biodiversity: adaptations, environments and responses to change, in: Oceanography and Marine Biology, edited by: Hawkins, S. J., Evans, A. J., Dale, A. C., Firth, L. B., and Smith, I. P., 1st Edn., CRC Press, https://doi.org/10.1201/9780429454455, 2018. a
Primpke, S., Wirth, M., Lorenz, C., and Gerdts, G.: Reference database design for the automated analysis of microplastic samples based on Fourier transform infrared (FTIR) spectroscopy, Anal. Bioanal. Chem., 410, 5131–5141, 2018. a
Reed, S., Clark, M., Thompson, R., and Hughes, K. A.: Microplastics in marine sediments near Rothera research station, Antarctica, Mar. Pollut. Bull., 133, 460–463, 2018. a
Revell, L. E., Kuma, P., Le Ru, E. C., Somerville, W. R. C., and Gaw, S.: Direct radiative effects of airborne microplastics, Nature, 598, 462–467, https://doi.org/10.1038/s41586-021-03864-x, 2021. a, b
Rios, L. M., Moore, C., and Jones, P. R.: Persistent organic pollutants carried by synthetic polymers in the ocean environment, Mar. Pollut. Bull., 54, 1230–1237, 2007. a
Ryan, P. G.: A Brief History of Marine Litter Research, in: Marine Anthropogenic Litter, edited by: Bergmann, M., Gutow, L., and Klages, M., Springer, Cham, https://doi.org/10.1007/978-3-319-16510-3_1, 2015. a
Seefeldt, M. W. and Cassano, J. J.: A description of the Ross Ice Shelf air stream (RAS) through the use of self‐organizing maps (SOMs), J. Geophys. Res.-Atmos., 117, D09112, https://doi.org/10.1029/2011JD016857, 2012. a
Shim, W. J., Hong, S. H., and Eo, S. E.: Identification methods in microplastic analysis: a review, Anal. Method., 9, 1384–1391, 2017. a
Stark, J. S., Corbett, P. A., Dunshea, G., Johnstone, G., King, C., Mondon, J. A., Power, M. L., Samuel, A., Snape, I., and Riddle, M.: The environmental impact of sewage and wastewater outfalls in Antarctica: An example from Davis station, East Antarctica, Water Res., 105, 602–614, 2016. a
Stein, A., Draxler, R. R., Rolph, G. D., Stunder, B. J., Cohen, M., and Ngan, F.: NOAA's HYSPLIT atmospheric transport and dispersion modeling system, Bull. Am. Meteorol. Soc., 96, 2059–2077, 2015. a, b
Suaria, G., Perold, V., Lee, J. R., Lebouard, F., Aliani, S., and Ryan, P. G.: Floating macro-and microplastics around the Southern Ocean: Results from the Antarctic Circumnavigation Expedition, Environ. Int., 136, 105494, https://doi.org/10.1016/j.envint.2020.105494, 2020. a
Tao, D., Zhang, K., Xu, S., Lin, H., Liu, Y., Kang, J., Yim, T., Giesy, J. P., and Leung, K. M.: Microfibers Released into the Air from a Household Tumble Dryer, Environ. Sci. Tech. Let., 9, 120–126, https://doi.org/10.1021/acs.estlett.1c00911, 2022. a
Tin, T., Lamers, M., Liggett, D., Maher, P. T., and Hughes, K. A.: Setting the Scene: Human Activities, Environmental Impacts and Governance Arrangements in Antarctica, in: Antarctic Futures, edited by: Tin, T., Liggett, D., Maher, P., and Lamers, M., Springer, Dordrecht, https://doi.org/10.1007/978-94-007-6582-5_1, 2014. a
Tin, T., Summerson, R., and Yang, H. R.: Wilderness or pure land: tourists’ perceptions of Antarctica, Polar J., 6, 307–327, 2016. a
Van Cauwenberghe, L., Vanreusel, A., Mees, J., and Janssen, C. R.: Microplastic pollution in deep-sea sediments, Environ. Pollut., 182, 495–499, 2013. a, b
Vandermeersch, G., Van Cauwenberghe, L., Janssen, C.R., Marques, A., Granby, K., Fait, G., Kotterman, M.J., Diogène, J., Bekaert, K., Robbens, J. and Devriese, L.: A critical view on microplastic quantification in aquatic organisms, Environ. Res., 143, 46–55, 2015. a
Waller, C. L., Griffiths, H. J., Waluda, C. M., Thorpe, S. E., Loaiza, I., Moreno, B., Pacherres, C. O., and Hughes, K. A.: Microplastics in the Antarctic marine system: an emerging area of research, Sci. Total Environ., 598, 220–227, 2017. a, b
Williamson, C. E., Neale, P. J., Hylander, S., Rose, K. C., Figueroa, F. L., Robinson, S. A., Häder, D.-P., Wängberg, S., and Worrest, R. C.: The interactive effects of stratospheric ozone depletion, UV radiation, and climate change on aquatic ecosystems, Photochem. Photobiol. Sci., 18, 717–746, 2019. a
Wright, S. L., Thompson, R. C., and Galloway, T. S.: The physical impacts of microplastics on marine organisms: a review, Environ. Pollut., 178, 483–492, 2013. a
Zhang, Y., Gao, T., Kang, S., Allen, S., Luo, X., and Allen, D.: Microplastics in glaciers of the Tibetan Plateau: Evidence for the long-range transport of microplastics, Sci. Total Environ., 758, 143634, https://doi.org/10.1016/j.scitotenv.2020.143634, 2021. a