the Creative Commons Attribution 4.0 License.
the Creative Commons Attribution 4.0 License.
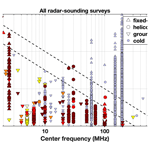
Brief communication: An empirical relation between center frequency and measured thickness for radar sounding of temperate glaciers
Joseph A. MacGregor
Michael Studinger
Emily Arnold
Carlton J. Leuschen
Fernando Rodríguez-Morales
John D. Paden
Radar sounding of the thickness of temperate glaciers is challenged by substantial volume scattering, surface scattering and high attenuation rates. Lower-frequency radar sounders are often deployed to mitigate these effects, but the lack of a global synthesis of their success limits progress in system and survey design. Here we extend a recent global compilation of glacier thickness measurements (GlaThiDa) with the center frequency for radar-sounding surveys. From a maximum reported thickness of ∼ 1500 m near 1 MHz, the maximum thickness sounded decreases by ∼ 500 m per frequency decade. Between 25–100 MHz, newer airborne radar sounders generally outperform older, ground-based ones. Based on globally modeled glacier thicknesses, we conclude that a multi-element, ≤30 MHz airborne radar sounder could survey most temperate glaciers more efficiently.
- Article
(823 KB) - Full-text XML
-
Supplement
(446 KB) - BibTeX
- EndNote
Measuring the thickness of Earth's mountain glaciers is essential for advancing understanding of their volume, flow and future amid ongoing anthropogenic warming, consequent mass loss and contribution to sea-level rise (e.g., Farinotti et al., 2019; Zemp et al., 2019). Radar sounding is unambiguously the preferred method for most surveys of glacier thickness (Welty et al., 2020). However, most mountain glaciers outside the polar regions are either observed or assumed to be temperate (i.e., at the pressure-melting point), and radar sounding of such ice is more challenging than for polar ice sheets (where most of the ice column is well below the pressure-melting point) or polythermal glaciers (e.g., Pritchard et al., 2020). Three main factors conspire to cause this challenge: (1) englacial water in pore spaces or fractures, increasing volume scattering (e.g., Fountain et al., 2005); (2) more common crevassing and supraglacial debris, increasing surface scattering (e.g., Herreid and Pellicciotti, 2020); and (3) warmer ice, increasing the englacial dielectric attenuation rate (e.g., Stillman et al., 2013).
Watts and England (1976) described what may be the primary challenge in radar sounding of temperate ice: meter-scale, water-filled englacial cavities that efficiently scatter incident radio waves where the ratio of those cavities' radius to the radar's englacial wavelength exceeds ∼ 0.1. Their analysis favored center frequencies 10 MHz to increase the signal-to-clutter ratio between the ice–bed reflection (signal) and any cavity-induced volume scattering (clutter). Their lucid description of this challenge motivated the development of numerous low-frequency radar sounders (e.g., Watts and Wright, 1981; Fountain and Jacobel, 1997; Conway et al., 2009; Mingo and Flowers, 2010; Rignot et al., 2013; Arnold et al., 2018; Björnsson and Pálsson, 2020). However, subsequent advances in available hardware, system design and processing demonstrated that higher-frequency (> 10 MHz) radar sounders can also sound hundreds of meters of temperate ice (e.g., Rutishauser et al., 2016; Langhammer et al., 2019; Pritchard et al., 2020). No synthesis yet exists of the success of these radar sounders as a function of center frequency, limiting our ability to identify outstanding opportunities in system and survey design for more efficient sounding of temperate glaciers. Here we evaluate past and potential radar-sounder performance by examining recent global compilations of both observed and modeled glacier thickness.
We primarily use three data sources in this study: (1) the maximum reported ice thickness for individual surveys compiled in the Glacier Thickness Database (GlaThiDa) version 3.1.0 (Welty et al., 2020); (2) the consensus modeled thickness estimates for all glaciers on Earth (Farinotti et al., 2019); and (3) the first-order regions, glacier locations, areas and identification numbers of the Randolph Glacier Inventory (RGI) version 6 (RGI Consortium, 2017).
We extend GlaThiDa with one additional field: the center frequency of the deployed radar sounder for surveys that used this method (Supplement). While englacial wavelength is likely the more fundamental physical property affecting radar-sounder success (Watts and England, 1976), it is the center frequency that is most often reported and straightforward to compile. In most cases, we could determine the value of this field directly from other metadata compiled by GlaThiDa. In dozens of cases, we reviewed the primary source for the survey to determine the center frequency, assuming that the deployed radar sounder with the lowest center frequency was that which detected the maximum ice thickness reported, if that was not stated explicitly. In 210 cases (4 % of radar-sounding surveys in GlaThiDa), we were unable to determine the center frequency.
For ground-based surveys, a potential source of ambiguity is whether the reported center frequency is for the antenna radiating in air or through ice, which can be challenging to determine from available metadata. The wavelengths radiated by a given antenna are determined by its physical size, design and input signal; these wavelengths are the same if that antenna is on the ground or airborne, but their frequencies will differ because the propagation velocity through air and ice differs. If the reported center frequency for a ground-based system is that for transmission through ice, then it will be lower by a factor of ∼ 1.79 (the radio-frequency index of refraction for ice) than that of most other reported frequencies (e.g., Mingo and Flowers, 2010), which are typically through air. Here we assume that all reported center frequencies are through air.
For all GlaThiDa entries that reported a maximum thickness for a presumed temperate glacier at the upper end of the range reported for that frequency, we reviewed the original study to validate the reported value. For all glaciers with raw glacier thickness data in GlaThiDa but no reported maximum value, which are mostly attributed to Rignot et al. (2013) and Rutishauser et al. (2016), we calculate the maximum thickness directly from those raw data. Finally, we adjust GlaThiDa's survey method field to further distinguish airborne radar-sounding surveys between helicopter and fixed-wing surveys.
We distinguish between regions that are most likely to contain temperate glaciers versus those that mostly contain polythermal or polar glaciers, while recognizing that substantial uncertainty remains in the thermal structure of many mountain glaciers (e.g., Wilson and Flowers, 2013). We assume that temperate glaciers are predominant within all RGI regions except Arctic Canada (03 and 04), Greenland (05), Svalbard (07), the Russian Arctic (09), and the Antarctic and sub-Antarctic (19). Glaciers situated at ≥ 67∘ latitude (e.g., McCall Glacier, Alaska, and Storglaciären, Sweden) and known polythermal glaciers in presumably otherwise temperate regions are excluded from the remaining set: (1) Hazard, Rusty and Trapridge glaciers, Canada (Narod and Clarke, 1980); (2) Gornergletscher, Switzerland (Rutishauser et al., 2016); and (3) Khukh Nuru Uul, Mongolia (Herren et al., 2013). In addition to the resulting 324 frequency–thickness pairs for glaciers in temperate regions from GlaThiDa, we also show maximum thicknesses from ground-based surveys recently completed for the Himalayas (440 m at 3.5 MHz; Pritchard et al., 2020), western Canada (318 m at ∼ 18 MHz in air; Pelto et al., 2020) and the Bagley Icefield, Alaska (1460 m at 1.875 MHz; Martin Truffer and John W. Holt, personal communication, 2020).
Figure 1a shows the relation between reported maximum ice thickness and radar-sounder center frequency, differentiated by survey year (if known or reported) and platform type (for presumed temperate glaciers in GlaThiDa: 155 ground-based, 102 helicopter and 67 fixed-wing). A general trend of decreasing measured thickness with increasing frequency is apparent. While qualitatively recognized by many practitioners and sometimes investigated directly for the purposes of system and survey design (e.g., Watts and Wright, 1981; Rutishauser et al., 2016; Pritchard et al., 2020), this relation has not been quantified previously across the full spectrum of frequencies used to sound terrestrial ice masses (∼ 1–1000 MHz).
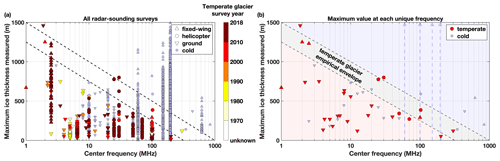
Figure 1(a) Synthesis of all GlaThiDa v3.1.0 reported maximum ice thicknesses versus the center frequency of the deployed radar sounder and (b) a subset showing the maximum value for each unique frequency. “Cold” glaciers are either those from the polar regions or those in presumed temperate regions that are known to be polythermal (blue symbols). Symbol shape indicates platform type. Black dashed lines represent the lower and upper bounds of the empirical envelope of apparent best sounding performance for temperate glaciers. In panel (a), for presumed temperate glaciers, symbol color indicates survey period (if reported). In panel (b), the maximum measured ice thickness for cold glaciers at four frequencies (60, 100, 150 and 195 MHz) exceeds the maximum value on the vertical axis (1500 m), represented by vertical blue arrows.
Most radar-sounding surveys of temperate glaciers before 2000 (55 of 63 surveys) deployed systems with frequencies below 20 MHz, while about half (138 of 258) of modern surveys (2000–onward) used higher frequencies (≥20 MHz). At very low frequencies (<3 MHz) and at higher ones (>20 MHz), it is these modern radar sounders that sound unusually large ice thicknesses compared to older surveys at nearby frequencies. However, between 3–20 MHz ground-based surveys are predominant and sound the thickest ice. Between 25–100 MHz, newer airborne systems tend to outperform ground-based systems at lower frequencies, and helicopter-borne systems tend to outperform fixed-wing systems (e.g., 100 MHz), presumably due to the former's slower platform speed and potentially lower altitude above ground level (Fig. 1b). Higher frequencies (≥ 60 MHz) can sound much thicker polythermal or polar ice than has been achieved for temperate glaciers, but this relative performance advantage for colder ice is reduced significantly below 60 MHz, which may be due to lower antenna gain and increased environmental interference.
The above synthesis contains multiple sampling biases. (1) A radar sounder can only sound temperate ice as thick as the glaciers it surveys, so reported thicknesses may underestimate an individual system's true capability. (2) Most surveys have rarely known beforehand where ice thickness is predicted to be greatest or its expected value, so for a given glacier that thickest location may not have been surveyed or a suitable radar sounder may not have been selected (this situation has ameliorated recently with the advent of globally modeled glacier thicknesses; e.g., Farinotti et al., 2019). (3) Ground-based surveys can only occur where it is safe to do so. (4) Some surveys were performed during the summer, when englacial water is likely more abundant and hinders radar performance. (5) Some reported center frequencies for ground-based surveys may be for ice, rather than for air as we have assumed (Sect. 2). (6) Some glaciers we assume are temperate – due to their regional setting and the lack of contraindicating observations – may not be temperate. The first five biases are likely negative; i.e., they induce an underestimate of the maximum ice thickness that could be sounded at a given frequency, while the sixth bias is positive with the opposite effect. Separately, off-nadir surface clutter is a well-known source of ambiguity in identification of the ice–bed reflection within valley glaciers (e.g., Holt et al., 2006). Most surveys had no direct method for surface-clutter discrimination (e.g., Conway et al., 2009; Rignot et al., 2013), but its bias can be positive or negative depending on glacier geometry and survey design.
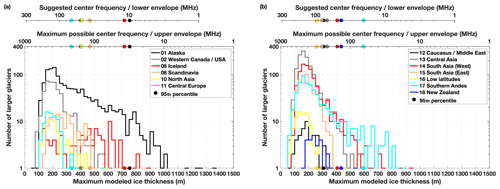
Figure 2Distribution (lines) and 95th percentile (circles) of maximum modeled ice thickness for all larger (≥5 km2) glaciers in RGI regions assumed to contain mostly temperate glaciers. RGI regions are separated into two panels for readability. Bin interval is 25 m. Upper horizontal axes are equivalent to the lower and upper bounds of the empirical envelope in Fig. 1, e.g., for a region whose 95th percentile maximum ice thickness is ∼ 500 m, the maximum center frequency of a suitable radar sounder for that region is ∼ 100 MHz, while ∼ 30 MHz is a more conservative suggested frequency.
Based on the above synthesis, we identify a simple envelope for the maximum temperate ice thickness sounded across the more than 2 decades of frequency spanned by deployed radar sounders (Fig. 1b). For the frequency range shown (1–1000 MHz), this empirical relation is , where Hmax is the maximum ice thickness in meters, is the maximum ice thickness in meters at 1 MHz and f is the center frequency in megahertz. This linear relation between ice thickness and logarithmic frequency was selected because it captures the predominant trend but does not overfit thickness maxima with as-of-yet unjustified complexity. While precise uncertainty bounds for this envelope cannot yet be specified, we present approximate lower and upper bounds for this envelope using values of 1250 and 1500 m, respectively, although we note that two surveys report sounding temperate ice slightly thicker than even the upper envelope suggests. The envelope's value lies in its indirect synthesis of the previously mentioned factors that challenge sounding of temperate ice, its identification of radar-sounder frequencies that may be performing near a natural or present technical limit (e.g., ∼ 2–3, ∼ 25–30 and 100 MHz) and others where radar sounders have either underperformed or may be unusually challenged by temperate ice (∼ 5–20 MHz).
Using this empirical envelope, we can then crudely relate the maximum modeled thickness distribution for each predominantly temperate RGI region to a suitable radar-sounder frequency (Fig. 2). We consider only larger glaciers (RGI-reported area ≥5 km2) that are more likely to be targeted for radar-sounding surveys. This comparison highlights the maximum possible frequency that could sound most glaciers in these regions under ideal conditions (upper bound of envelope) and the more conservative suggested frequency (lower bound), both of which are larger than recommended previously (Watts and England, 1976). For most temperate regions (10 of 13), this analysis suggests that a modern ≤100 MHz radar sounder could potentially sound the maximum thickness of 95 % of their glaciers (<500 m; Fig. 2), although a ≤30 MHz system is a more conservative suggestion. For thicker glaciers in Alaska, Iceland and the southern Andes, a lower-frequency (≤ 30 MHz) radar sounder remains necessary for many glaciers, and ≤ 10 MHz may be more suitable, as observed in practice (e.g., Conway et al., 2009; Björnsson and Pálsson, 2020).
We note two contrasting caveats to this analysis. (1) While the modeled maximum thicknesses do not appear to be biased significantly relative to measured maxima ( m for the 36 % of GlaThiDa surveys we could confidently match using glacier names to a modeled glacier in the RGI inventory), it is not uncommon for radar-sounding surveys of ice masses to report ice thicknesses greater than predicted beforehand, so any survey design based on Fig. 2 should assume a negative model bias and favor the lower envelope. (2) The maximum modeled thickness is only reached at a single point on a glacier, so surveys aiming to measure glacier volume must also consider the ability to resolve smaller thicknesses at a satisfactory resolution. This trade-off could favor a higher center frequency, for which a larger bandwidth is easier to achieve, potentially resulting in a finer range resolution.
Our analysis of GlaThiDa-compiled thicknesses challenges the conventional wisdom that only low-frequency (≤10 MHz) radar sounders are suitable for sounding temperate glaciers that are hundreds of meters thick (Fig. 1). An empirical envelope derived from this synthesis suggests that – for most temperate glaciers on Earth – higher frequencies are indeed appropriate for radar sounding thereof, assuming suitable system design (Fig. 2). For example, this envelope suggests a higher upper limit (∼ 30 MHz) on potentially suitable radar sounders for sounding temperate ice up to ∼ 750 m thick (Fig. 1), but a more conservative interpretation still suggests that ∼ 500 m is possible at this frequency. Advances in hardware and system design could further increase this range, e.g., solid-state transmit–receive switches, higher peak-transmit powers and platform-aware numerical optimization of antenna configurations (Arnold et al., 2020). While a full accounting of the physical underpinnings of the empirical envelope we identified was beyond the scope of this study, the envelope cannot be explained by the relatively weak dispersion of the radio-frequency dielectric attenuation rate (MacGregor et al., 2015), so volume and surface scattering are the more likely controls.
This empirical envelope can help further balance a radar-sounding survey's scientific objectives with the system to be deployed. For example, selecting a system toward the lower end of frequency range considered (e.g., ≤ 10 MHz) is only necessary if the objective is to sound the thickest temperate ice in a handful of regions. Alternatively, a higher-frequency (≥ 30 MHz) radar sounder could be deployed to more finely resolve glacier volume at the possible expense of not sounding the thickest portions of some glaciers. Not all glaciers are created equal, and in some cases an exceptionally crevassed surface, thicker supraglacial debris or abundant englacial water will continue to necessitate the use of lower frequencies to ensure successful sounding.
Models of glacier thickness increasingly incorporate mass conservation and global satellite remote-sensing datasets, but there remains an outstanding need for additional thickness measurements to both validate those models and refine their underlying assumptions (e.g., Farinotti et al., 2019; Pelto et al., 2020). Even the most spatially extensive ground-based radar-sounding survey of a glacierized region can be easily dwarfed by a single airborne survey – assuming that survey's radar sounder can match the performance of the ground-based system (e.g., Pritchard et al., 2020). Langhammer et al. (2019) demonstrated recently that two orthogonal pairs of helicopter-towed 25 MHz antennas can better sound temperate glaciers because the sum of the antenna response is less sensitive to unfavorable bed geometry. Alternatively, a slightly higher frequency (e.g., 30 MHz) translates to a dipole antenna that is ≤ 5 m long, a sufficiently small dimension that several such elements could be mounted on a fixed-wing aircraft (e.g., Arnold et al., 2018). More than two such antenna elements in a plane perpendicular to the platform's direction of travel are essential to resolving cross-track ambiguity in the direction of arrival of coherently recorded reflections, i.e., “swath mapping” (e.g., Holschuh et al., 2020). By transforming some of what is presently discarded as noise (discontinuous near-bed subsurface reflections not attributable to surface clutter) into useful signal (geolocated, off-nadir ice–bed reflections), swath mapping could substantially expand our ability to measure glacier thickness efficiently.
The Supplement associated with this article contains the new metadata generated by this study in a format similar to GlaThiDa v3.1.0. The analysis was performed using MATLAB R2021a and both its Mapping and Statistics & Machine Learning toolboxes. The script used to perform the analysis and generate the figures is available at https://doi.org/10.5281/zenodo.4895135 (MacGregor, 2021).
The supplement related to this article is available online at: https://doi.org/10.5194/tc-15-2569-2021-supplement.
JAM initiated this study, led the analysis and drafted the manuscript. MS aided in the study design, analysis and manuscript preparation. EA, CJL, FRM and JDP aided in the analysis and manuscript preparation.
Joseph A. MacGregor is a member of the editorial board of this journal.
We thank the authors of and contributors to GlaThiDa, Farinotti et al. (2019) and the RGI Consortium for making our study possible through open distribution of well-documented, homogenized datasets. We thank Richard Hale, John W. Holt, Hester Jiskoot, Horst Machguth, Laurent Mingo and Martin Truffer for valuable discussions. We thank scientific editor Daniel Farinotti, Melchior Grab and the anonymous reviewer for constructive reviews that improved this paper.
This research has been supported by the NASA/GSFC Strategic Science program.
This paper was edited by Daniel Farinotti and reviewed by Melchior Grab and one anonymous referee.
Arnold, E., Rodriguez-Morales, F., Paden, J., Leuschen, C., Keshmiri, S., Yan, S., Ewing, M., Hale, R., Mahmood, A., Blevins, A., Mishra, A., Karidi, T., Miller, B., and Sonntag, J.: HF/VHF radar sounding of ice from manned and unmanned airborne platforms, Geosciences, 8, 182, https://doi.org/10.3390/geosciences8050182, 2018.
Arnold, E., Leuschen, C., Rodriguez-Morales, F., Li, J., Paden, J., Hale, R., and Keshmiri, S.: CReSIS airborne radar and platforms for ice and snow sounding, Ann. Glaciol., 61, 58–67, https://doi.org/10.1017/aog.2019.37, 2020.
Björnsson, H. and Pálsson, F.: Radio-echo soundings on Icelandic temperate glaciers: history of techniques and findings, Ann. Glaciol., 61, 25–34, https://doi.org/10.1017/aog.2020.10, 2020.
Conway, H., Smith, B., Vaswani, P., Matsuoka, K., Rignot, E., and Claus, P.: A low-frequency ice-penetrating radar system adapted for use from an airplane: test results from Bering and Malaspina Glaciers, Alaska, USA, Ann. Glaciol., 50, 93–97, https://doi.org/10.3189/172756409789097487, 2009.
Farinotti, D., Huss, M., Fürst, J. J., Landmann, J., Machguth, H., Maussion, and Pandit, A.: A consensus estimate for the ice thickness distribution of all glaciers on Earth, Na. Geosci., 12, 168–173, https://doi.org/10.1038/s41561-019-0300-3, 2019.
Fountain, A. G. and Jacobel, R. W.: Advances in ice radar studies of a temperate alpine glacier, South Cascade Glacier, Washington, U.S.A., Ann. Glaciol., 24, 303–308, https://doi.org/10.3189/S0260305500012350, 1997.
Fountain, A. G., Jacobel, R. W., Schlichting, R., and Jansson, P.: Fractures as the main pathways of water flow in temperate glaciers, Nature, 433, 618–621, https://doi.org/10.1038/nature03296, 2005.
Herreid, S. and Pellicciotti, F.: The state of rock debris covering Earth's glaciers, Nat. Geosci., 13, 621–627, https://doi.org/10.1038/s41561-020-0615-0, 2020.
Herren, P.-A., Eichler, A., Machguth, H., Papina, T., Tobler, L., Zapf, A., and Schwikowski, M.: The onset of Neoglaciation 6000 years ago in western Mongolia revealed by an ice core from the Tsambagarav mountain range, Quaternary Sci. Rev., 69, 59–83, https://doi.org/10.1016/j.quascirev.2013.02.025, 2013.
Holschuh, N., Christianson, K., Paden, J., Alley, R. B., and Anandakrishnan, S.: Linking postglacial landscapes to glacier dynamics using swath radar at Thwaites Glacier, Antarctica, Geology, 48, 268–272, https://doi.org/10.1130/G46772.1, 2020.
Holt, J. W., Peters, M. E., Kempf, S. D., Morse, D. L., and Blankenship, D. D.: Echo source discrimination in single-pass airborne radar sounding from the Dry Valleys, Antarctica: Implications for orbital sounding of Mars, J. Geophys. Res., 111, E06S24, https://doi.org/10.1029/2005JE002525, 2006.
Langhammer, L., Rabenstein, L., Schmid, L., Bauder, A., Grab, M., Schaer, P., and Maurer, H.: Glacier bed surveying with helicopter-borne dual-polarization ground-penetrating radar, J. Glaciol., 65, 123–135, https://doi.org/10.1017/jog.2018.99, 2019.
MacGregor, J.: joemacgregor/temperate_sounding: published version (Version v1.0), Zenodo [code], https://doi.org/10.5281/zenodo.4895135, 2 June 2021.
MacGregor, J. A., Li, J., Paden, J. D., Catania, G. A., Clow, G. D., Fahnestock, M. A., Gogineni, S. P., Grimm, R. E., Morlighem, M., Nandi, S., Seroussi, H., and Stillman, D. E.: Radar attenuation and temperature within the Greenland Ice Sheet, J. Geophys. Res.-Earth, 120, 983–1008, https://doi.org/10.1029/2014JF003418, 2015.
Mingo, L. and Flowers, G. E.: An integrated lightweight ice-penetrating radar system, J. Glaciol., 56, 709–714, https://doi.org/10.3189/002214310793146179, 2010.
Narod, B. B. and Clarke, G. K. C.: Airborne UHF radio echo-sounding of three Yukon glaciers, J. Glaciol., 25, 23–31, https://doi.org/10.3189/S002214300001025X, 1980.
Pelto, B. M., Maussion, F., Menounos, B., Radíc, V., and Zeuner, M.: Bias-corrected estimates of glacier thickness in the Columbia River Basin, Canada, J. Glaciol., 66, 1051–1063, https://doi.org/10.1017/jog.2020.75, 2020.
Pritchard, H., King, E. C., Goodger, D. J., McCarthy, M., Mayer, C., and Kayastha, R.: Towards Bedmap Himalayas: development of an airborne ice-sounding radar for glacier thickness surveys in High-Mountain Asia, Ann. Glaciol., 61, 35–45, https://doi.org/10.1017/aog.2020.29, 2020.
RGI Consortium: Randolph Glacier Inventory – A Dataset of Global Glacier Outlines: Version 6.0: Technical Report, Global Land Ice Measurements from Space, https://doi.org/10.7265/N5-RGI-60, 2017.
Rignot, E., Mouginot, J., Larsen, C. F., Gim, Y., and Kirchner, D.: Low-frequency radar sounding of temperate ice masses in Southern Alaska, Geophys. Res. Lett., 40, 5399–5405, https://doi.org/10.1029/2013GL057452, 2013.
Rutishauser, A., Maurer, H., and Bauder, A.: Helicopter-borne ground-penetrating radar investigations on temperate alpine glaciers: A comparison of different systems and their abilities for bedrock mapping, Geophysics, 81, WA119–WA129, https://doi.org/10.1190/GEO2015-0144.1, 2016.
Stillman, D. E., MacGregor, J. A., and Grimm, R. E.: The role of acids in electrical conduction through ice, J. Geophys. Res.-Earth, 118, 1–16, https://doi.org/10.1029/2012JF002603, 2013.
Watts, R. D. and England, A. W.: Radio-echo sounding of temperate glaciers: ice properties and sounder design criteria, J. Glaciol., 17, 39–48, https://doi.org/10.3189/S0022143000030707, 1976.
Watts, R. D. and Wright, D. L.: Systems for measuring thickness of temperate and polar ice from the ground or from the air, J. Glaciol., 27, 459–469, https://doi.org/10.3189/S0022143000011485, 1981.
Welty, E., Zemp, M., Navarro, F., Huss, M., Fürst, J. J., Gärtner-Roer, I., Landmann, J., Machguth, H., Naegeli, K., Andreassen, L. M., Farinotti, D., Li, H., and GlaThiDa Contributors: Worldwide version-controlled database of glacier thickness observations, Earth Syst. Sci. Data, 12, 3039–3055, https://doi.org/10.5194/essd-12-3039-2020, 2020.
Wilson, N. J. and Flowers, G. E.: Environmental controls on the thermal structure of alpine glaciers, The Cryosphere, 7, 167–182, https://doi.org/10.5194/tc-7-167-2013, 2013.
Zemp, M., Huss, M., Thibert, E., Eckert, N., McNabb, R., Huber, J., Barandun, M., Machguth, H., Nussbaumer, S. U., Gärtner-Roer, I., Thomson, L., Paul, F., Maussion, F., Kutusov, S., and Cogley, J. G.: Global glacier mass changes and their contributions to sea-level rise from 1961 to 2016, Nature, 568, 382–386, https://doi.org/10.1038/s41586-019-1071-0, 2019.