the Creative Commons Attribution 4.0 License.
the Creative Commons Attribution 4.0 License.
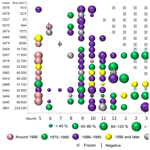
Warming temperatures are impacting the hydrometeorological regime of Russian rivers in the zone of continuous permafrost
Olga Makarieva
Nataliia Nesterova
David Andrew Post
Artem Sherstyukov
Lyudmila Lebedeva
Large Arctic river basins experience substantial variability in climatic, landscape, and permafrost conditions. However, the processes behind the observed changes at the scale of these basins are relatively poorly understood. While most studies have been focused on the “Big 6” Arctic rivers – the Ob', Yenisey, Lena, Mackenzie, Yukon, and Kolyma – few or no assessments exist for small and medium-sized river basins, such as the Yana and Indigirka River basins. Here, we provide a detailed analysis of streamflow data from 22 hydrological gauges in the Yana and Indigirka River basins with a period of observation ranging from 35 to 79 years up to 2015. These river basins are fully located in the zone of continuous permafrost. Our analysis reveals statistically significant (p<0.05) positive trends in the monthly streamflow time series during the autumn–winter period for most of the gauges. The streamflow increases in a stepwise pattern (post-1981) for 17 out of 22 gauges in September (average trend value for the period of record is 58 % or 9.8 mm) and 15 out of 22 gauges in October (61 % or 2.0 mm). The positive trends are seen in 9 out of 19 rivers that do not freeze in November (54 %, 0.4 mm) and 6 out of 17 rivers that do not freeze in December (95 %, 0.15 mm). Precipitation is shown to decrease in late winter by up to 15 mm over the observational period. Additionally, about 10 mm of precipitation that used to fall as snow at the beginning of winter now falls as rain. Despite the decrease in winter precipitation, no decrease in streamflow has been observed during the spring freshet in May and June in the last 50 years (from 1966); moreover, five gauges show an increase of 86 % or 12.2 mm in spring floods via an abrupt change in 1987–1993. The changes in spring freshet start date are identified for 10 gauges; the earlier onset in May varies from 4 to 10 d over the observational period. We conclude that warmer temperatures due to climate change are impacting the hydrological regime of these rivers via changes in precipitation type (rain replacing snow). Other factors, such as the melting of permafrost, glaciers, and aufeis or changes in groundwater conditions, are likely to contribute as well; however, no direct observations of these changes are available. The changes in streamflow can have a significant impact on the ecology of the zone of continuous permafrost, while the increasing freshwater fluxes to the Arctic Ocean can impact the Arctic thermohaline circulation.
- Article
(14431 KB) - Full-text XML
-
Supplement
(1911 KB) - BibTeX
- EndNote
Numerous studies have shown that river streamflow in northern Eurasia and North America is increasing (Holland et al., 2007; Shiklomanov and Lammers, 2013; Rawlins et al., 2010). Most of them are focused exclusively on the “Big 6” Arctic rivers – the Ob', Yenisey, Lena, Mackenzie, Yukon, and Kolyma (Peterson et al., 2002; Holmes et al., 2013; Rood et al., 2017). Large Arctic river basins are characterized by a great variety of climatic, landscape, and permafrost conditions, and reported streamflow changes are not homogeneous in terms of different runoff characteristics and timescales. The mechanisms of the observed changes can hardly be understood on the large scale of the Big 6.
Compared to large rivers, the changes in flow in small and medium rivers in cold regions have been studied much less. Tananaev et al. (2016) found that 30 small and medium-sized rivers out of 100 in the Lena River basin showed trends in mean annual flow. Significant changes have been recently reported for small and middle-sized rivers in northwestern Canada (Spence et al., 2011), Alaska (Stuefer et al., 2017), and the Canadian High Arctic (Lamoureux and Lafrenière, 2018) and attributed to climate change and permafrost disturbances. Further analysis of small river basins could reveal the mechanisms behind ongoing changes, as at larger scales attributing changes can be problematic.
River runoff change estimates in northeastern Siberia are limited and contradictory. Magritsky et al. (2013) reported an increase in the total runoff of Yana and Indigirka during the period 1976–2006 by 1.5 %–3 % compared to the period before 1976 and noted that the runoff of these rivers increased in summer and autumn by 20 %–25 % and did not change in winter. According to Georgievsky (2016), on the contrary, there is an increase in Yana and Indigirka River winter runoff by 40 % over the period 1978–2012 compared with the period 1946–1977 and an increase in the spring flood. Majhi and Yang (2011) concluded that the Yana River monthly flow increases at the Jubileynaya gauge (224 000 km2) for the period of 1972–1999 for June, August, September, October, and April, while May, July, and March monthly flow have decreased. Bring and Destouni (2014) reported an absence of any significant changes in the Yana and Indigirka streamflow accompanied by a decrease in precipitation of up to 38 mm for the period 1991–2002 compared to 1961–1991.
While some studies have examined flows at the outlet of the Yana and Indigirka River basins, estimations of streamflow changes in small and medium-sized rivers in the Yana and Indigirka River basins do not exist. The objective of this research is therefore a quantitative assessment of current changes in hydrometeorological regime within two large Arctic river basins, with both basins completely located within the continuous permafrost zone, which have long-term runoff observations along the main rivers and their tributaries at smaller scales.
In Sect. 2 of this paper, we will present the study area. In Sect. 3, we will describe the data to be examined and the methods that we will use. Results of the analysis will be presented in Sect. 4, while these results will be put into context and potential causal factors influencing the results described in Sect. 5. Finally, some conclusions will be drawn in Sect. 6.
2.1 General description, relief
The Yana and Indigirka rivers are two of the few large Arctic rivers whose basins are completely located in the zone of continuous permafrost (Fig. 1). The terrain is mainly mountainous. There is high elevation in the Verkhoyansk (Orulgan, 2389 m), Chersky (Pobeda, 3003 m), and Suntar–Khayata (Mus-Khaya, 2959 m) ranges, as well as wide river valleys. The average altitude of the research basins ranges from 320 to 1410 m and the elevations of the outlet gauges range from −1.55 to 833 m, respectively. The lower parts of the Yana and Indigirka basin area are represented by the Yano–Indigirskaya Lowland. The average height of the lowland is above 30–80 m, with some parts of the lowland raised up to more than 500 m.
2.2 Climate
The study territory is the region where the Northern Hemisphere's “pole of cold” is located. The absolute minimum there has reached record levels for the Northern Hemisphere: down as far as −71 ∘C in Oymyakon and −68 ∘C in Verkhoyansk (Ivanova, 2006). The research region's climate is distinctly continental. Long-time average annual air temperature ranges from −16.1 ∘C (Oymyakon, 726 m, 1930–2012) to −13.1 ∘C (Vostochnaya, 1288 m, 1942–2012). Minimum mean monthly temperatures are typically observed in January and can fall as low as −47.1 ∘C (Oymyakon) and −33.8 ∘C (Vostochnaya). Maximum average monthly temperatures are observed in July, averaging 15.7 and 11.8 ∘C for stations Yurty (590 m, 1957–2012) and Vostochnaya, respectively. Steady cold weather begins in the first week of October; spring starts in the second part of May or at the beginning of June, when seasonal snow cover starts to melt. Snow accumulation is relatively small and accounts for 25–30 cm of depth. Annual average precipitation at the Verkhoyansk meteorological station (137 m, 1966–2012) is about 180 mm, while at Vostochnaya (1966–2012) it is 280 mm. Most precipitation (over 60 %) occurs in summer, with a peak in July of up to 70 mm per month (Vostochnaya, 1966–2012).
2.3 Soil, vegetation, and landscapes
The research basins are situated in the transitional zone between forest–tundra and coniferous taiga (Fig. 2). For high-altitude mountain areas above 1900 m a.s.l., goltsy (bald mountain) and small glaciers with minimum and maximum areas from 0.024 to 5.76 km2 (GLIMS and NSIDC, 2005) are typical. Vegetation in this landscape is absent. Broken stone is present in the form of glacial frost-split boulders as well as diluvia soil of the valley slopes with admixed loam material. Below is the tundra, which is characterized by the distribution of a tight and depressed layer of grass and moss with bushes, under which there is rock formation and some ice with admixtures. Most of the research areas are covered by larch woodland with moss–lichen cover. In river valleys, grass–moss larch forest and swampy sparse growth forest are typical. Soil types at these landscapes are clayey podzol with partially decayed organic material underlain by frozen soil and bedrock. The updated map of permafrost landscapes (Fedorov et al., 2018) can be used for a more detailed description of the studied basins.
2.4 Permafrost
In the studied river basins, permafrost thickness can reach over 450 m at watershed divides and up to 180 m in river valleys and depressions. There are highly dynamic cryogenic features such as retrogressive thaw slumps found in the Yana River basin that indicate ongoing permafrost degradation processes (Günther et al., 2015). Permafrost temperature at a depth of zero annual amplitude in the studied region typically varies from −3 to −11 ∘C and the active layer depth is from 0.3 to 2 m (Explanatory note to the geocryological map of the USSR, 1991).
2.5 Hydrological regime and water balance
The hydrological regime is characterized by spring freshet, high summer–autumn rainfall floods, and low winter flow. In winter, small and medium-sized rivers freeze thoroughly. Spring freshet starts in May–June (on average 20 May) and lasts approximately for a month and a half. In summer, glacier runoff, melting aufeis, and snowfields contribute to streamflow.
The amount of precipitation at meteorological stations varies from 176 mm yr−1 (ID 24261) to 279 mm yr−1 (ID 24679). At the high-altitude area, annual precipitation can reach up to 600 mm or more per year. Average annual precipitation at Suntar–Khayata station (2068 m) in 1957–1964 reached 690 mm with corrections for wind and wetting losses (Grave et al., 1964). The annual precipitation amount at the mountain peaks is estimated to be as high as 800 mm (Vasiliev and Torgovkin, 2002). The average annual flow at studied rivers varies from 58 mm (ID 3433, 18.3 km2) to 362 mm (ID 3516, 16.6 km2). This difference of a factor of 6 at two watersheds of similar area shows the impact of local conditions on runoff formation. Annual values of flow at the outlet gauges of the Indigirka (ID 3871, 305 000 km2) and Yana (ID 3861, 224 000 km2) rivers are 166 and 156 mm, respectively. According to observational data (Makarieva et al., 2018a) annual evapotranspiration depends on the type of underlying surface and the distribution of landscapes over the catchment area. On average, it ranges from 70 mm in the goltsy landscape to 140 mm where there is sparse growth of larch trees (Lebedeva et al., 2017).
2.6 Groundwater and taliks
Since the Yana and Indigirka River basins are located in continuous permafrost, groundwater can be found in a seasonally developed active layer, underneath permafrost (supra-permafrost water), and in taliks (Shepelev, 2011). Depending on texture, infiltration, and other soil properties, the active layer stores and transmits water to rivers in summer and early autumn. Water in the active layer could sustain recession river flow in autumn only until the freezing front reaches the permafrost table. Taliks with thicknesses of several meters typically exist under small and middle-sized rivers even if the rivers freeze in winter (Mikhailov, 2013). Through-taliks are typically found along the river channels with water depth exceeding 3–5 m. Rivers in continuous permafrost often lose water to channel taliks during summer and gain in winter (Arzhakova, 2001). Through-taliks are also associated with fractured deposits, with depth exceeding the permafrost thickness (Glotov et al., 2011). Rivers with lengths >800 km and basin area >75 000 km2 typically do not freeze up and continue to flow over the winter. Year-round groundwater springs and winter flow of large rivers suggest a supra-permafrost water contribution.
2.7 Aufeis and glaciers
Aufeis (naleds), which forms at mountain foothills, as well as in submountain and intermountain depressions, is another distinguishing feature of the region. According to Simakov and Shilnikovskaya (1958), the research area had 1281 aufeis fields covering an area of about 2082 km2. In winter, the aufeis reduces both river and underground flow, and in the warm season, melted aufeis waters form an additional source of river runoff. Most flow from melting aufeis is observed in May–June. In most cases, the share of the aufeis component does not exceed 3 %–7 % of annual river runoff (Reedyk et al., 1995; Sokolov, 1975), but in May its proportion may exceed 50 % of the total runoff (Sokolov, 1975).
Few glaciers are found in the research area (GLIMS and NSIDC, 2005). The total area of glaciers is about 2.2 km2 at the Yana River and 436 km2 (0.14 % of the area) of the Indigirka River basin. At some studied basins, glaciers reach up to 0.38 % of the basin area (ID 3488, Indigirka River – Yurty). The glacier runoff may exceed 3.8 % of the overall annual streamflow and reach 6.1 % of streamflow in July and August, for example at the basin of the Agayakan River (the Indigirka basin), where glaciers cover over 1.35 % of the catchment. On the slopes of the Suntar–Khayata and Chersky ranges, perennial snowfields and rock glaciers are widespread (Lytkin and Galanin, 2016). They, along with the ice of the active layer and summer atmosphere precipitation, may represent a significant source of flow in local rivers; however, in this respect they are poorly studied (Zhizhin et al., 2012; Lytkin and Galanin, 2016).
3.1 Data
Daily discharge series for 22 hydrological gauge stations of the Russian Hydrometeorological Network in the Yana and Indigirka River basins were analyzed. Most of the stations have hydrological data until 2014–2015, but two of them ceased operation in 2007 and one (river Indigirka – Vorontsovo, ID 3871) in 1996. The median length of the observation series is 62 years with minimum and maximum values reaching 36 and 80 years, respectively (Table 1, Fig. 1). A total of 10 out of the 22 catchments examined here have areas less than 10 000 km2, with five of them being less than 100 km2. Maximum catchment area is 305 000 km2 (ID 3871). The average elevation above sea level of the examined catchments varies from 320 m (ID 3433) to 1410 m (ID 3499). Thus, the study covers not only large, but also small and medium-sized rivers over a broad range of elevations and landscapes typical for the studied mountain region.
We used the values of monthly and annual flow (mm), and spring freshet start dates (counting days from the beginning of the year) used in the analysis were estimated based on daily discharge data for the entire observation period from 1936 up to and including 2014–2015. There are different ways to determine a freshet start date. For example, Lesack et al. (2013) defined the initiation of freshet discharge based on a threshold value of 3 % increase in discharge per day. In this study, wherein nonfreezing and freezing rivers were investigated, a different approach was considered to be more appropriate. A day was defined as a freshet flood start date if its discharge reached or exceeded 20 % of the average discharge value in the studied year. All the streamflow series were visually checked for the adequacy of this assumption.
The uncertainties associated with discharge determination change significantly from year to year and strongly depend on the computational methods used and the frequency of discharge measurements. Possible errors in flow values shown in the database as reliable are as follows for all gauges: average annual flow errors do not exceed 10 %, and monthly flows errors are 10 %–15 % for the open channel period and 20 %–25 % for winter months. The errors of “approximate” flow, placed in the database in parentheses, can exceed the values indicated above by 2–3 times (State water cadastre, 1979). The errors for streamflow in large rivers may be slightly lower: monthly flow errors are 4 %–12 % for the open channel period and 17 % for winter months in the Lena River basin (Shiklomanov et al., 2006). Daily air temperature and precipitation data series, observed at 13 weather stations (the elevation range varies from 20 to 1288 m) located in the studied basins over different periods from 1935 (but not later than 1966) to 2015 (some stations to 2012), were reduced to average monthly value series (Table 2). Monthly soil temperature under natural cover at different depths down to 320 cm from three weather stations over a period of 1966–2015 was also analyzed. A detailed description of soil temperature data sets and their quality control methods may be found in Sherstyukov and Sherstyukov (2015). Active layer thickness (ALT), used for the analysis, was estimated with polynomial interpolation of soil temperatures at depth (Streletskiy et al., 2015). The sources of the data are described in Makarieva et al. (2018b).
3.2 Methods
In this study, we applied a combination of widely used statistical methods for trend detection and assessment of their values in hydrometeorological data. General guidance with a detailed description of different types of tests and their adequate application can be found in Kundzewicz and Robson (2004). Time series of runoff characteristics (monthly flow, estimated flood starting dates, and maximum daily flows) and meteorological elements (monthly and annual values of air temperature and precipitation; soil temperature and ALT) were evaluated for stationarity in relation to the presence of monotonic trends with Mann–Kendall and Spearman rank-correlation tests at the significance level of p<0.05 (Mann, 1945; Kendall, 1975; Lehmann, 1975). If both tests suggested a trend at the significance level p<0.05, a serial correlation coefficient was tested at unity lag r(1). With the serial correlation coefficient r<0.20, the trend was considered reliable. In the case of r≥0.20, to eliminate serial correlation in the input series the “trend-free pre-whitening” procedure (TFPW), described by Yue et al. (2002), was carried out. “Whitened” time series were repeatedly tested with a Mann–Kendall nonparametric test at the significance level p<0.05. The Pettitt test (Pettitt, 1979) was applied to look for the presence of a change point in time series at p≤0.05; the Buishand range test (Buishand, 1982) was used to search for numerous discontinuities at the same significance level. Trend values were estimated with the Theil–Sen estimator (Sen, 1968) and are given in the relevant data units along with the percentage change since the beginning of observations. Note that the trends are presented for the entire period of observations and not for the period after the change point was identified, as there can be multiple trends within the period of observations. In some (specified) cases, the significance level was relaxed to the value p>0.05.
The results of the trend analysis are presented in Tables 3–8. Multidimensional tables with colorful designations showing not only the trend values but also presenting the distribution of change points across the basins can be found in the Supplement (Tables S1–S5). We used the same colors as in Tables S1–S5 in Fig. 3, showing the percentage change in monthly and annual streamflow at different change point periods, and Figs. 4 and S12–S17, which present the spatial patterns of the change points in different months.
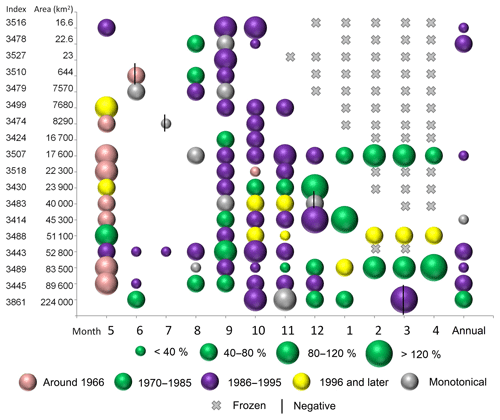
Figure 3Change in monthly streamflow represented as a percent, along with the period in which that change occurred. Data are for both Yana and Indigirka River basins and are sorted in order of basin area.
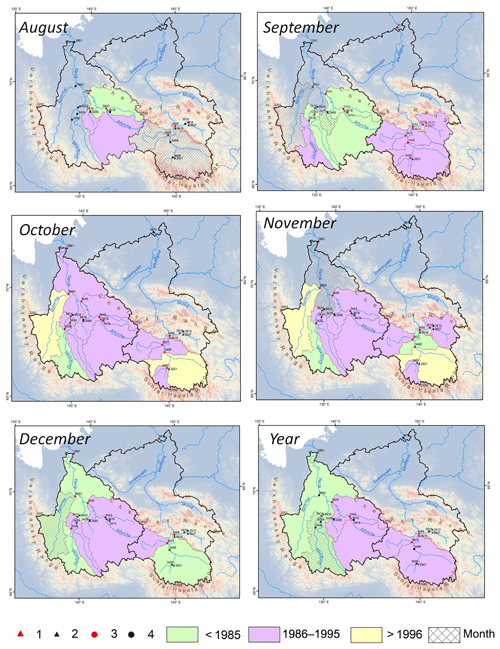
Figure 4The periods of changes in streamflow in August, September, October, November, December, and annually. Red and black indicate the presence and absence of the trends, respectively. The triangles and circles indicate the basin area of less and more than 1000 km2, respectively. Detailed figures for each month can be found in Figs. S13–S18.
4.1 Air temperature
The increase in annual air temperature is statistically significant at all 13 studied stations (Tables 3, S1, Fig. S1) with an average cumulative value of about +2.1 ∘C (from +0.16 to +0.46 and average +0.35 ∘C per 10 years) for the historical period of observations (minimum, maximum, and mean number of years correspond to 47, 80, and 63).
Table 3Changes in monthly and annual air temperature (∘C).
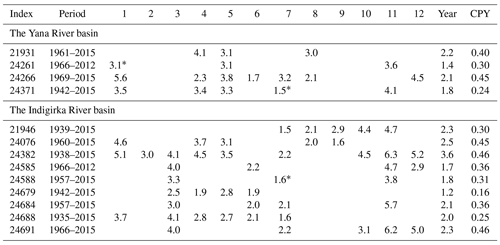
Most of the values have statistical significance of p<0.05. The asterisk (*) indicates the significance . CPY (∘C (10 yr)−1) is for average change in temperature per 10 years.
During the period April to July, there is a significant air temperature trend at most of the weather stations of the region; average trend values in these months account for +3.0, 3.1, 1.9, and 2.3 ∘C (Table 3). A positive air trend is observed in March in the mountainous part of the Indigirka River (five stations with elevation >500 m). In August, air temperature has increased at three Yana basin stations and two lowland stations in the Indigirka basin, accounting on average for a 3.4 and 2.4 ∘C increase, respectively. In September, air temperature has increased at two stations in the lower part of the Indigirka River basin by 2.3 ∘C on average. There are no significant trends in October at the Yana basin weather stations, but the mean positive trend at five stations of the Indigirka basin accounts for 3.7 ∘C in the same month. In November, at 7 stations out of 13 across both basins, positive trends are statistically significant and reach on average 4.7 ∘C with a maximum value of 6.3 ∘C at Delyankir (ID 24691). Positive winter air temperature trends are significant at four weather stations in December, six in January, and at one station in February. In March, the positive trend value is 3.6 ∘C on average for seven meteorological stations in the mountainous part of the Indigirka River basin. Change point analysis was conducted for 9 stations out of the 13 with full data series. Almost all changes occur through abrupt shifts rather than monotonic trends. Most change points are attributed to two periods: 1975–1985 and 1986–1996. The shifts in winter air temperature occurred at the beginning of the millennium (1999–2004).
4.2 Precipitation
Monthly precipitation analysis for 13 meteorological stations in the region, located at elevations from 20 to 1288 m (1966–2015, some stations until 2012), has shown no evidence of a systematic positive trend (Tables 4, S2, Fig. S2).
Table 4Changes in monthly, seasonal, and annual precipitation (millimeters and percent), 1966–2015.
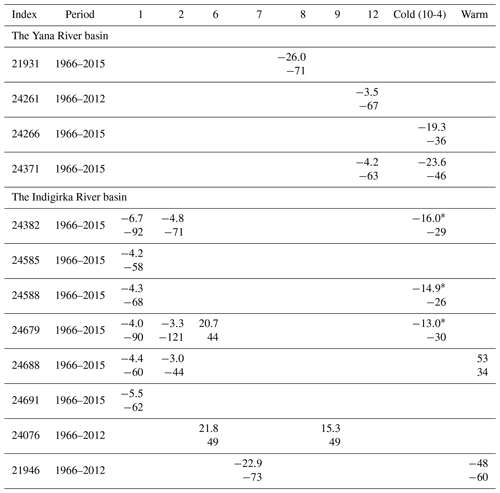
Only the columns corresponding to the months in which the trends of precipitation were detected are shown in the table. Most of the values have statistical significance of p<0.05. The asterisk (*) indicates the significance . The first and second figures represent the mean trend values since the beginning of observations (millimeters and percent).
In contrast, at six weather stations out of nine in the Indigirka basin, a statistically significant precipitation decrease is observed in January and at three stations in February. In the Yana basin, two stations have shown a decrease in precipitation in December. The average statistically significant decrease in winter months ranges from −3.0 to −6.7 mm per month or −44 % to 92 %. In lowland areas that are close to the furthest downstream gauges of the Yana and Indigirka rivers, reliable negative trends were identified in July (−73 %, −23 mm) at Chokurdakh (ID 21946, the Indigirka basin) and in August (−71 %, −26 mm) at Kazachie (ID 21931, the Yana basin). It is unlikely that runoff from these areas significantly impacts the flow at the outlets of the Yana and Indigirka rivers as most of the runoff is produced in the upper, mountainous parts of the basins. Positive trends in summer months are observed at two stations of the Indigirka basin: +44 % (21 mm) at Vostochnaya (ID 24679) in June and +49 % (22 mm) and +49 % (15 mm) in June and September at Deputatsky (ID 24076) (Table 4). We evaluated cold season precipitation calculated as the sum from October to April. Two stations in the Yana River basin have shown a statistically significant (p<0.05) decrease of about 40 % or 20 mm. Three stations in the Indigirka River basin experienced negative trends at the level of significance with a reduction of precipitation about −28 % or 15 mm per season. The change points for solid precipitation are estimated to occur between 1980 and 1996 with most of them occurring in 1986. A positive trend in warm period precipitation (May–September) is detected at Oymyakon (ID 24688), in the upstream parts of the Indigirka River, with values of 35 % or 54 mm. Warm season precipitation has decreased by almost half (−48 % or −60 mm) at the outlet of the Indigirka River (Chokurdakh, ID 21946).
Rain–snow precipitation ratio
The state and timing of precipitation in May and September (which are the months when the 0 ∘C air temperature threshold is crossed) were analyzed. The results show that meteorological stations in the higher elevations (which are mostly located in the Indigirka River basin) exhibit a shift towards larger amounts of rain rather than snow in both studied months. In May, three stations show an upward trend for a larger fraction of rain (p<0.05). Vostochnaya (ID 24679, 1288 m) exhibits the strongest changes: the mean share for the whole period is 0.43, with a trend value of 0.45. Oymyakon and Agayakan (IDs 24684, 24688) have a mean value of rain share equal to 0.79 and on average increase by 0.16 (Table 5). In September, the mean share of rain fluctuates from 0.56 at Vostochnaya (ID 24679, 1288 m) to 0.79 at Agayakan and Oymyakon (IDs 24684, 24688; 726 and 776 m). The trend value varies from 0.15 to 0.19 for increases in rain share for the whole period of observation at five stations (Table 5). In absolute values, the amount of rain increased on average by 60.7 %, or 12.2 mm in total, during the same period at six stations. Pettitt and Buishand tests for change points indicate an abrupt shift of precipitation regime in September in 1991–1993 for four stations.
4.3 Soil temperature and active layer thickness (ALT)
There are three meteorological stations with soil temperature data available for the entire historical period, including the beginning of the 21st century, with a total area of 529 000 km2: two in the Indigirka and one in the Yana River basin. We analyzed soil temperature at a depth of 80 cm and estimated maximum active layer thickness (Table 6). An analysis of changes in the number of days with positive soil temperature at the depth of 80 cm for 2001–2015 in comparison with the long-term mean value for 1971–2000 was also carried out. Positive soil temperatures at the depth of 80 cm at the described weather stations are observed from July to October.
Table 6Changes in soil temperature at 80 cm of depth and maximum active layer thickness (ALT).
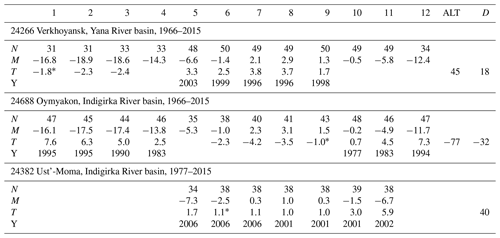
* N – the number of values in the analyzed series; M, ∘C – mean temperature; T, ∘C1 – temperature trend Sen's estimate; Y – change point (year); ALT (cm) – change in maximum active layer thickness; D – change in the number of days with positive soil temperature at the depth of 80 cm, 2001–2015, compared to the long-term mean value for 1971–2000. Most of the values have statistical significance of p<0.05. The asterisk (*) indicates the significance .
The Yana River basin (Verkhoyansk, ID 24266, 137 m a.s.l.). Significant positive soil temperature trends at the depth of 80 cm in summer (from May to September) and negative ones in winter (from January to March) have been identified (Table 6, Fig. S3). On average, soil temperature at the studied depth increased by +3.4 ∘C in summer months for the last 50 years. Maximum trend values are observed in July and August and account for +4.1 and 4.4 ∘C, respectively. In winter, the corresponding temperature average dropped by −2.2 ∘C for the same period of observations. Winter trends should be viewed with some caution as there was a significant gap in the observations from 1985 to 1999. A change point estimated with Pettitt's test was identified around 1996–1999 in June–September and 2003 in May. Accordingly, ALT (mean 170 cm) increased by 45 cm. It increased for 10 years (1997 to 2007) and was then stable for the next 8 years. The number of “warm” days at the depth of 80 cm has increased, on average, by 18 d over the last 15 years in comparison with the long-term mean value for 1971–2000 from 87 to 105 cm.
The Indigirka River basin (Oymyakon, ID 24688, 726 m a.s.l.). In the uppermost part of the Indigirka River basin, significant negative soil temperature trends at the depth of 80 cm in summer are observed, along with positive trends in winter (Table 6, Fig. S3). In June–September, soil temperature at 80 cm of depth dropped on average by −2.8 ∘C and increased by +4.8 ∘C in October–April. The change in soil temperature occurs in an abrupt manner – the shifts in winter temperature are identified in 1977 (October), 1983 (November, April), 1990 (May), and 1994–1995 (December–February). In summer, the shift cannot be estimated due to the data gap from 1990 to 1999.
Due to the temperature decrease in summer, estimated ALT (mean 165 cm) dropped by 77 cm over the period 1966–2015 (−1.5 cm a−1). The number of warm days has dropped by 32 d (from 103 to 71) over the period 2000–2015 in comparison with the previous 30-year period.
The Indigirka River basin (Ust'-Moma, ID 24382, 196 m a.s.l.). Statistically significant positive trends are identified for the period from May to November, and on average temperature increases by +2.1 ∘C over 39 years (1977–2015) (Table 6, Fig. S3). The largest trends are observed in October and November and account for +3.0 and +5.9 ∘C, respectively. The change points are estimated as occurring in 2001–2002 for August–November and 2006 for May–July. The trend in ALT (mean 127 cm) is insignificant. The number of warm days has increased, on average, by 39 d from 1977 to 2015 (from 57 to 96).
4.4 Streamflow
Positive statistically significant trends (p<0.05) in monthly streamflow were identified during two main periods: in autumn–winter and in the first month of a spring flood – in May for most of the river gauges (Fig. 3, Tables 7–8, S3–S4). Most of the time series with significant trends are nonstationary, and changes are attributed to break points. Single and double change points are common for studied streamflow records and are described below.
4.4.1 Spring flow (May and June)
In May, a statistically significant increase in streamflow is observed at 12 of the 21 studied gauges. These 12 may be divided into two groups. The first group (Fig. S4, Group A) contains seven gauges with basin areas from 8290 to 89 600 km2 characterized by a longer continuous series of observations beginning between 1937 and 1956. The shifts of streamflow occur in 1964–1966 and follow a similar behavior. In most cases, a negative tendency changes to an insignificant positive one. Average trend rates are 79 % or 8.9 mm for this group. If the assessment of trend is made for the last 50 years after the change point (1966–2015), no significant trend is detected in May for this group of gauges. The second group (Fig. S5, Group B) consists of five gauges with no significant trend, followed by break points during the period from 1980 to 1999 and positive trends thereafter varying from 5.5 to 25.7 mm with an average value of 12.2 mm (or from 64 % to 103 %, 86 % on average). Minimum basin area for this group is 16.6 km2, and the maximum is 52 800 km2. No trends were identified in May at the Yana and Indigirka River outlet gauges for the periods 1972–2007 (ID 3861, 224 000 km2) and 1936–1996 (ID 3871, 305 000 km2), respectively. In June, a statistically significant (p<0.05) positive trend in streamflow is observed at four gauges of the Yana River (Fig. S6). One gauge exhibits a monotonic increase in flow in June for the last 50–60 years and the other three gauges have break points in 1983–1995. The average positive trend value is 40 % or 16.4 mm. A small river in the Indigirka basin (ID 3510, 644 km2; p<0.057) has shown a total decreased trend value during the last 70 years with a break point in 1967. The streamflow shifts from a high mean with a negative tendency to a low mean with a slightly positive tendency. If the analysis is made for the period 1967–2015, streamflow in June at this gauge does not present any detectable changes. The total negative trend value accounts for −61 % or −14.2 mm.
4.4.2 Summer–autumn floods (July–September)
In July, no statistically significant changes in streamflow occur in the Indigirka River basin. In the Yana River basin, two nested gauges show opposite tendencies (Tables 7–8, S3–S4). The streamflow at gauge ID 3474 (8290 km2) monotonically decreased by 38 % (26 mm). At the same, time gauge ID 3443 (52 800 km2) experienced a total increase in streamflow of 17 mm or 36 % with a break point in 1995. In August, an increase in streamflow was identified at 7 out of 22 studied gauges with an average increase of 55 % or 18 mm. The areas of the catchments where discharge increases in August vary from 644 to 89 600 km2. Two nested gauges (IDs 3507, 3489) have a monotonic increasing trend from the mid-1950s to 2015. The others, including two nested gauges of the Adycha River (IDs 3443, 3445), exhibit the shift in 1982–1987 (Figs. S6, 2).
Table 7Changes in monthly and annual streamflow (millimeters and percent) and freshet onset dates. The Yana River basin.
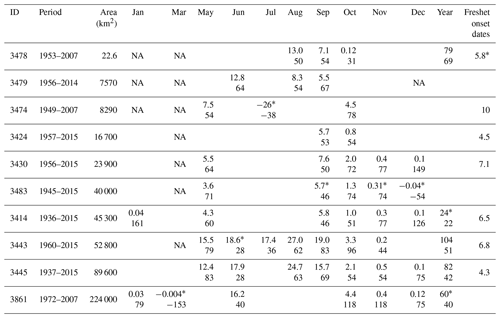
Only the columns corresponding to the months in which the trends of flow were detected are shown in the table. Most of the values have statistical significance of p<0.05. The asterisk (*) indicates the significance . The first and second figures indicate the mean trend value (millimeters and percent). NA means that there is no flow in this month.
In September, positive trends were identified at 17 out of 22 gauging stations (average value is 58 % or 9.8 mm); three of those rivers are small ones (catchment areas are less than 100 km2), and the others are medium and large rivers (Tables 7–8, S3–S4, Figs. S7, 2). This assessment includes a positive trend identified at the Indigirka River outlet gauge (ID 3871) for the shorter period 1936–1996. Some river gauges of the Yana River basin (IDs 3478, 3479, 3483) have shown a monotonic increase, and others have change points mainly in the 1981–1982 period (nested gauge IDs 3414, 3424, 3443, and 3445); one gauge (ID 3430) exhibited a shift of mean in 1994. In the Indigirka River basin, seven gauges have a change point in 1992–1993; two of them with a time series length of about 70 years have additional change points in 1965. The Indigirka River gauge (ID 3871) with the data series interrupted in 1996 has a change point in 1965 as well. One may assume that this gauge would show a positive shift in September around 1993 as did the others of its tributaries if longer observational data were available.
4.4.3 Low flow (October–March)
In October, streamflow increases at 15 out of 22 gauges (on average by 61 % or 2.0 mm) and in November at 11 out of 17 nonfrozen gauges (on average by 54 % or 0.4 mm) (Tables 7–8, S3–S4, Figs. S7–S8). Most of the changes are abrupt. In October, most of the step trends occur in 1987–1993 with several exceptions in 1982 and 2001–2002; in November, they occur in 1981–1999, mainly around 1994. In December, positive trends are found at 6 out of 15 nonfrozen gauges (Tables 7–8, S3–S4, Figs. S8, 2). The average positive trend magnitude is 95 % or 0.15 mm. Change points occur from 1981 to 1994. A negative monotonic trend in December is found at gauge 3483 with the magnitude −54 % or −0.04 mm. A decrease in streamflow at the Indigirka River at Vorontsovo (ID 3871) is identified in December and January for the period 1936–1996 and accounts for 32 % and 25 % or −0.20 and −0.08 mm, respectively, with change points in 1964–1969 (Figs. S8–S9, 2). In contrast, at the Yana River at the Yubileynaya gauge (ID 3861), streamflow increases by 74 % (0.12 mm) monotonically in December and by a step trend in January 1981, amounting to 77 % (0.03 mm). A positive trend is also found in the upstream part of the Yana River (ID 3414) in January with a magnitude of 161 % or 0.04 mm in 80 years (change point in 1977) (Figs. S9, 2). In February, no trends are found at the Yana River basin where only five gauges stay unfrozen in this month of the year (Tables 7, S3). In March, the streamflow trend at the Yana River at Yubileynaya (ID 3861) is negative with a change point around 1989 when the monthly average value of 0.003 mm declines to zero. Positive trends are identified at two gauges of the Indigirka River (IDs 3488, 3489) and one of its main tributaries, the Elgi River (ID 3507), with a mean magnitude of 74 % (0.07 mm), 83 % (0.02 mm), and 87 % (0.08 mm) in February, March, and April, respectively (Tables 8, S4, Fig. S9). The change points are 1981–1987 for the ID 3489 and ID 3507 gauges and 2002–2004 for the ID 3488 gauge in those 3 months.
4.4.4 Annual flow
In the Yana River basin, statistically significant changes in annual streamflow are found at the Adycha River tributary. Maximum percentage and net flow changes accounting for +51 % or +104 mm during 1960–2015 are observed at the Adycha River in Ust'-Charky (ID 3443) with a basin area of 51 100 km2 and a step change in 1995. The change point at the nested lower gauge ID 3445 (basin area 89 600 km2) occurred in 1987 with a total increase in flow of 42 % or 82 mm for the period from 1937 to 2015 (Tables 7, S3, Fig. S10).
An increasing annual trend is also found at gauges (ID 3478, 22.6 km2; ID 3479, 7570 km2) with an increase of 73 % or 79 mm with a shift in 1988 and monotonic growth by 48 % or 34 mm. Monotonic flow changes are observed at two nested gauges of the Yana River (ID 3414, 45 300 km2; ID 3861, 224 000 km2) with magnitudes of 22 % (24 mm) and 39 % (60 mm) (Tables 7, S3, Fig. S10).
In the mountainous part of the Indigirka River basin, positive step trends in annual streamflow are found starting from 1993 at two gauges in the upstream of the Indigirka River (IDs 3488, 3489) and its tributary, the Elgi River (ID 3507), with an average magnitude of 27 % or 49 mm. An increase in annual streamflow is also observed at the smallest basin from the analyzed set (ID 3516, 16.6 km2) with a magnitude of 31 % or 115 mm. Runoff in August and September have contributed the most of all months to the annual streamflow rate changes at these gauging stations (Table 8, Fig. S10).
4.4.5 Maximum daily streamflow
An analysis of maximum daily streamflow was carried out for the warm period from May to September. In general, the patterns of changes in maximum daily discharges replicate the change in monthly streamflow (Table S1). The main changes are observed in May with break points around 1966, when negative trends change into an insignificant positive one. The percentage change in May for eight gauges averages 69 % over the whole period of observations. In September, the break points in terms of maximum discharge occur around 1993 in the Indigirka and in 1976–1981 in the Yana River basin. The average increase in maximum discharge in September reaches up to 55 % for 15 gauges.
4.4.6 Freshet onset dates
In the rivers with a basin area less than 2000 km2, freshet starts between 11 and 18 May; for the larger rivers this date shifts to the end of the month (25 May, on average). Freshet starts 4–8 d earlier at the end of the time series compared to 50–70 years ago (the trends are statistically significant) in eight rivers with identified streamflow changes in May, as well as in three gauges for which monthly streamflow increase in May is statistically insignificant (Tables 7–8, S3–S4); two of these gauges have catchment areas smaller than 1000 km2 (Table 1). In the Indigirka River basin, three gauges have change points of freshet onset dates in 1967 and another three show a monotonic shift. In the Yana River basin, four gauges show a monotonic change to an earlier onset, with one gauge having a change point in 1978 and two in 1995–1997.
5.1 Air temperature
Global land-surface air temperature has increased over the period 1979–2012 by 0.25–0.27 ∘C per 10 years (IPCC, 2014). According to Jamalov et al. (2012) the warmest decade in Russia was 1990–2000, while the highest temperature anomaly was recorded in 2007 (temperature anomaly of +2.06 ∘C), followed by 1995 (anomaly of +2.04 ∘C) and 2008 (anomaly of +1.88 ∘C). The Arctic has warmed at more than twice the global rate over the past 50 years. The greatest increase of more than 2 ∘C since 1960 occurred during the cold season (AMAP, 2017). Data from our study support these observations. Mean annual air temperature (MAAT) increases in the Yana and Indigirka River basins by about +2.1 ∘C (1966–2015). The values of the trends assessed in this study (from +0.16 to +0.46 ∘C per 10 years) slightly exceed other observations. Interpolated MAAT trends between 1956 and 1990 in the studied region are from 0.15 to 0.30 ∘C per 10 years (Romanovsky et al., 2007). Kirillina (2013) reported an air temperature increase at the Verkhoyansk, Ust'-Moma, and Oymyakon meteorological stations from 1 to 2 ∘C in the warm season and from 1.6 to 1.9 ∘C in the winter season for the period 1941–2010. The different periods analyzed could partly explain the difference in estimated trends. As noted by Fedorov et al. (2014) and Kirillina (2013), there were several phases with different air temperature tendencies for continental regions of northeastern Siberia in the 20th and 21st centuries. The last warming phase started in the 1960s or 1970s and was preceded by 3 or 4 decades of cooling. The lowest trends of 0.16 to 0.30 ∘C per 10 years were identified at the stations with the longest MAAT time series that partly included the cooling phase: Ust'-Charky (ID 24371, 1942–2015), Chokurdakh (ID 21946, 1939–2015), Vostochnaya (ID 24679, 1942–2015), and Oymyakon (ID 24688, 1935–2015). It suggests that the actual MAAT trend for last 40 years is spatially homogenous for the Yana and Indigirka River basins. Trend values exceeding 0.03 ∘C yr−1 are slightly higher than the global average and agree with other regional estimations of 0.03–0.05 ∘C yr−1 (Pavlov and Malkova, 2009).
5.2 Precipitation
Although precipitation is projected to increase over the pan-Arctic basin, this is not always supported by ground meteorological data (Rawlins et al., 2010). Small and insignificant positive trends from 0.63 to 5.82 mm yr−1 are reported for the 1951–2008 period for the high latitudes of the Northern Hemisphere (60 to 90∘ N; IPCC, 2014). Pan-Arctic cold season precipitation (October–May) increased by 3.6 mm per decade (1.5 % per decade) over the period 1936–2009 (AMAP, 2017; Callaghan et al., 2011). Kirillina (2013) reported that precipitation in the region shows a positive trend with the exception of the Oymyakon meteorological station.
The presented analysis for 1966–2015 (2012 at some stations) has shown no evidence of a systematic positive trend in annual precipitation and even displays a negative trend of solid precipitation for several stations, including Verkhoyansk (ID 24266). Savelieva et al. (2000) reference the decrease in winter precipitation over the territory of eastern Siberia to changes in the location and intensity of the Siberian High and Aleutian Low before and after the 1970s. The complex topography of the Arctic region may cause a considerable underestimation of precipitation as meteorological stations tend to be located in low-elevation areas (Serreze et al., 2003). Analyzed precipitation data did not undergo wind correction, which varies from 10 % in summer to 80 %–120 % in winter due to the effect of wind on gauge undercatch of snowfall (Yang et al., 2005). This study therefore confirms the high uncertainty of the spatial pattern of precipitation trends in cold regions (Hinzman et al., 2013).
5.3 Soil temperature
Permafrost temperatures have risen in many areas of the Arctic (AMAP, 2017). Long-term data on permafrost temperature from boreholes for the Yana and Indigirka basins are extremely scarce. Romanovsky et al. (2010) reported an increase in mean annual ground temperature (MAGT) in the eastern part of northern Yakutia (including the Yana and Indigirka River basins) of up to 1.5 ∘C over the last 20 years at 15 m of depth. Pavlov and Malkova (2009) reported annual ground temperature linear trends of 0.02–0.03 ∘C yr−1 for northeastern Russia. Air temperature increases in the May–July period at Oymyakon (ID 24688); however, soil temperature dropped on average by −2.8 ∘C (1966–2015) in summer. We suggest that the data for Oymyakon are not reliable as the decrease in soil temperature occurred in an abrupt manner, which appears to be related to the replacement of an instrument (Fig. S11). Additionally, it is hypothesized that the increase in liquid precipitation should warm permafrost through advective heat transport.
Identified trends of air, soil temperature, and precipitation at the Verkhoyansk (ID 24266) and Ust'-Moma (ID 24382) stations agree with each other. At Verkhoyansk, a soil temperature increase in May–September follows an air temperature upward tendency in April–August with a 1-month delay. The soil temperature drop in winter may be caused by a decrease in snow depth (Sherstukov, 2008) due to a statistically significant decrease in precipitation in the cold season from October to April (Table 4). At Ust'-Moma, the soil temperature increase from May to November could be explained by a statistically significant air temperature rise for 9 months out of 12.
Detected trends from −0.05 to +0.12 ∘C yr−1 at 80 cm of depth in different months for 1966 (1977) to 2015 at Verkhoyansk and Ust'-Moma show significant scatter compared to reported trends for the northeastern regions that vary from 0 to 0.03 ∘C yr−1. In eastern Siberia and the Russian Far East, ALT generally increased between 1996 and 2007 but has since been more stable (AMAP, 2017), which is confirmed by the temporal patterns of ALT change at Verkhoyansk (ID 24266).
5.4 Streamflow
Streamflow significantly (p<0.05) increased at least in one month at 19 of the 22 analyzed gauges. Three small rivers (ID 3501, 84.4 km2, 1120 m, 98 mm; ID 3480, 98 km2, 570 m, 81 mm; ID 3433, 18.3 km2, 320 m, 58 mm) do not show any significant changes in any studied streamflow metrics.
Analysis of the runoff data for the Yana and Indigirka River basins has shown statistically significant positive discharge trends in May and the autumn–winter period for the last few decades (accompanied by significant warming). Statistically significant increases are seen in 12 out of 22 gauges in May, 17 out of 22 in September, 15 out of 22 in October, 9 out of 19 in November, 6 out of 17 in December, 4 out of 12 in January, 3 out of 8 in February, and 3 out of 7 in March. Note that the total number of gauges decreases below 22 in the period from November to April because the rivers freeze.
5.4.1 Autumn
September shows the most considerable change in hydrological regime. The increase in streamflow happened at basins of all sizes in 17 of the 22 studied gauges. In September, air temperature increased only at 2, and precipitation increased only at 1 meteorological station out of 13.
In the Indigirka River basin, the increase in streamflow can be attributed to the shift of precipitation type in September. Investigating the correlation between monthly streamflow and precipitation in September for four small watersheds (area <100 km2) with meteorological stations located nearby, we found that the correlation coefficient varies from 0.16 to 0.59 for liquid precipitation and from 0.14 to 0.60 for total precipitation (Table 9, Fig. S12). We could not explain the reasons for low correlation at gauge ID 3433 (Table 9). It is also worth noting that for gauge ID 3501, the correlation coefficient increases from 0.27 to 0.46 for total and liquid precipitation, respectively.
Table 9Correlation coefficient of monthly streamflow and total and liquid precipitation in September at small watersheds.
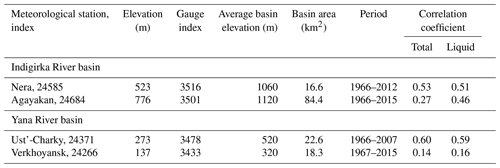
The shift of precipitation from solid to liquid occurs starting from 1993 and manifests in a considerable increase in rain fraction in comparison with snow at the stations located in the mountains. The fact that the changes in streamflow are observed regardless of basin size and in general match the break points of precipitation ratio shift indicates that it is climate rather than other possible factors (permafrost thaw, increased groundwater connectivity, etc.) that drives those changes. The average increase in streamflow reaches up to 9.8 mm, which is comparable with the mean absolute increase in rain precipitation of 12.2 mm. In the Yana River basin, one would expect a similar linkage between precipitation and streamflow in autumn, but this cannot be confirmed due to the lack of meteorological stations at higher elevations. Spence et al. (2011) have shown that the trend towards more autumn rainfall in the northwestern sub-Arctic Canadian Shield, with no increase in total precipitation, has been sufficient to cause late season peaks in discharge and higher winter low flows. Recessional curves of early autumn flood events extend later into the autumn and winter seasons. The streamflow changes in October generally repeat the spatial pattern of changes in September but with lower magnitude. The hypothesis of Berghuijs et al. (2014), stating that a shift from a snow-dominated towards a rain-dominated regime would likely reduce streamflow, is contradicted by the results of this study for the rivers in the continuous permafrost zone.
5.4.2 Winter
Upward trends in low flow are observed at Mackenzie River (Yang et al., 2015), at the Lena River and its tributaries (Tananaev et al., 2016), and in most of the Arctic (Rennermalm et al., 2010). The widely accepted hypothesis is that increased low flow indicates permafrost degradation, aquifer activation, and better connectivity between surface and subsurface water. Increasing active layer thickness, enlarged infiltration, and subsurface water contribution to winter discharge by deeper and longer flow pathways sustain increasing winter streamflow in permafrost environments (Karlsson et al., 2015; St. Jacques and Sauchyn, 2009; Tananaev et al., 2016).
Change points for low flow were detected in the 1980s, 1990s, and 2000s. Similar change points are observed at the Lena River basin (Tananaev et al., 2016), where the major shifts in all nonstationary time series occur in the 1990s, but when the data from adjacent decades are combined, the major changes in minimum discharge occur between 1985 and 1995 and in mean annual daily flow between 1995 and 2005.
The changes in November–December tend towards basins larger than 17 000 km2, although many smaller ones do not freeze up until January. Glotova and Glotov (2015) attribute that to the fact that the fraction of groundwater contribution to middle-sized and large basins is higher than that to smaller ones in the continuous permafrost zone.
Gurevich (2009) and Dzamalov and Potehina (2010) have substantiated the hypothesis that river ice cover regulates groundwater feeding into rivers in cold regions. The hypothesis is that in colder winters, with a significant thickness of ice, the total water discharge decreases in small river basins. In less severe winters, when the thickness of ice is lower, the underground contribution to streamflow in the river is higher by the end of winter.
Shiklomanov and Lammers (2014) report significant negative linear trends for the outlet gauges of the Lena, Yenisey, and Yana rivers where decreases in maximum ice thickness over 1955–2012 reached up to 73, 46, and 33 cm, respectively. Average values of maximum ice thickness for the same rivers were about 180, 105, and 153 cm, respectively, for the period 1955–1992. Several 10 d series of river ice depth for the Lena River at Tabaga for the period 1955–2015 were analyzed based on a Mann–Kendall test at the significance level of p<0.05. Negative statistically significant trends were identified in March and April with a Theil–Sen estimator. The depletion of river ice depth intensifies from March to April and decreases by 27 to 49 cm, or 20 %–35 % (project C3S_422_LOT1_SMHI; Copernicus Climate Change Service, 2017–2019). However, Shiklomanov and Lammers (2014) have found that the relationship between annual maximum ice thickness and mean river discharge over the November–April period has shown no significant correlation: the highest correlation coefficients were found for the Yenisey () and Lena rivers ().
5.4.3 Freshet
The increases in monthly streamflow in May occur in an abrupt manner. At 7 gauges (area ≥8290 km2) out of 12, the shift of flow happened around 1966 and was accompanied by two consequent years (1967, 1968) with extraordinarily high streamflow in May. Monthly flow in May 1967 and 1968 exceeded the monthly average by 3.8–6.5 times. Moreover, at 9 gauges out of 22 it was the highest flow through the whole period of observations. At the other six gauges, streamflow was the second highest among the observations with three of them differing by less than 5 % from the historical maximum and the other three from 11 % to 27 % lower.
No significant trends were detected for the period after the change point (1967–2015). Changes in spring freshet start date were identified at 10 gauges with streamflow changes in May. Trend value varies from 4 to 10 d earlier. This agrees with Savelieva et al. (2000), who stated that the final frost in spring has been 12–15 d earlier than the mean value in Yakutia. Smith (2000) estimated the time shift of the start date of spring ice cover events for the outlet of the Indigirka River at Vorontsovo (ID 3871) as 8.1 d for the period of observations (1937–1992).
Another five basins have shown the shift of flow in May during the period from 1980 to 1999. In four cases out of five, that shift can be attributed to an earlier freshet. Yang et al. (2015) documented increases in May flows for the Mackenzie and Yukon rivers, related to earlier melting of snow. An earlier start of freshet agrees with identified significant air temperature increases in May at 9 out of the 13 meteorological stations in the region. However, in contrast to the Lena River basin, where strong warming in spring led to an advance of the snowmelt season into late May and resulted in a lower daily maximum discharge in June (Yang et al., 2002; Tan et al., 2001), at the study gauges, streamflow has not been reduced in June (except ID 3510).
5.4.4 Maximum flow
According to recent projections, the annual maximum river discharge could almost double by the mid-21st century at the outlets of major Siberian rivers (Shkolnik et al., 2017). Existing assessments of maximum flow changes across cold regions do not fully confirm future projections. Shiklomanov et al. (2007) found no widespread significant change in spring maximum discharge, except for the Lena River, among the 139 gauging stations in the Russian Arctic. Tananaev et al. (2016) reported trends in maximum discharge only in 9 time series (negative at three and positive at six gauges) out of the 105 in the Lena River basin. The Ob' and Lena rivers showed uncorrelated changes in spring peak with those aggregated from small natural watersheds located within these larger river basins (Shiklomanov et al., 2007). There are significant negative trends (p<0.1) over 1950–2001 from aggregated maximum discharge for the Kolyma River basin (Shiklomanov et al., 2007). The outcomes from this study support these results; we found no significant changes in maximum flow in any of our analyzed river basins.
5.5 Water balance
5.5.1 Precipitation and streamflow relationship
Positive discharge trends in the Arctic have been established in recent years (McClelland et al., 2006; Peterson et al. 2002, 2006; Shiklomanov and Lammers, 2013). Increasing precipitation has been suggested as the one of the drivers of increasing streamflow (Dyurgerov and Carter, 2004; McClelland et al., 2004). Bring et al. (2016) project long-term streamflow increases over the Yana and Indigirka rivers by 50 % during the 21st century by combining information from global climate model runs of multiple scenarios. The findings of this study largely contradict this, with an increase in streamflow reported at most of the gauges despite no substantial change in the absolute amount of precipitation. We estimate that 10 mm of snow is lost in September as rain draining directly into streamflow. Adding the decrease in precipitation in February–March, the decrease in snow water equivalent during winter may reach up to 15–20 mm in total. Curiously, though, no evident decrease in streamflow is observed during freshet or summer months.
Inconsistent trends in discharge and precipitation have also been reported for other Arctic rivers. In some cases there is an opposite sign in the direction of runoff changes from that in precipitation (Karlsson et al., 2015). Berezovskaya et al. (2004) have shown that the patterns in increasing trends of runoff from Siberian rivers cannot be resolved from the apparent lack of consistent positive trends in the considered precipitation datasets.
Milliman et al. (2008) suggest that a fluctuating climate in the Yana River watershed precluded delineating a statistically significant change in either precipitation or discharge, but concluded that the Indigirka River basin had a statistically significant decrease in precipitation and nonsignificant increase in runoff.
5.5.2 Evapotranspiration
The issue of increasing streamflow in the absence of increasing precipitation may be explained by decreased evapotranspiration. By increasing the active layer depth and thus potentially lowering the water table, evapotranspiration might decrease, leading to increases in runoff (McClelland et al., 2004). Milliman et al. (2008) proposed that decreased evapotranspiration due to earlier snowmelt and changes in water storage within the drainage basins could be a potential source of excess streamflow. Based on modeling results, Stieglitz et al. (2003) showed that as warming occurs mostly during winter months it does not lead to an increase in evapotranspiration.
On the other hand, Rawlins et al. (2010) argue that evapotranspiration is increasing. This is supported by reanalysis data for the pan-Arctic domain. According to model simulations, evapotranspiration has a significant trend of 0.11 mm yr−2 (Variable Infiltration Capacity model; 1950–1999), while annual total evapotranspiration from land surface models (LSMs) also shows a positive trend (0.40 mm yr−2) (Rawlins et al., 2010). A longer growing season, observed as a result of climate warming in the 20th century, is likely to result in continued upward trends in evapotranspiration (Huntington, 2004).
5.6 Cryosphere
5.6.1 Thawing permafrost
Permafrost temperature in Russia has been increasing and the active layer has been deepening for the last 20–30 years (Romanovsky et al., 2010; Sherstyukov and Sherstyukov, 2015). Subsurface ice melting along with air temperature increase, widespread over the studied area (Brown et al., 1997; Yang et al., 2002), can contribute to the streamflow increase (Frey and Mclelland, 2009). Though McClelland et al. (2004) argue that if water released from thawing permafrost was making a significant contribution to the observed increase in annual discharge from Eurasian Arctic rivers, one might expect that watersheds with the most permafrost cover would show the largest increase in runoff, but no such pattern is apparent.
An analysis using satellite-based gravimetry at the Lena basin suggests that the storage of water in these areas has increased over the past decades (Velicogna et al., 2012; Muskett and Romanovsky, 2009), but the causes of this phenomenon are not clear (Velicogna et al., 2012). One reason may be permafrost degradation because warming in the active layer will cause greater connectivity between surface and subsurface water (Walvoord and Kurylyk, 2016) and talik development (Yoshikawa and Hinzman, 2003; Jepsen et al., 2013). Most groundwater discharge occurs through areas overlying open taliks, so they play the important hydrogeological role of accommodating preferential pathways acting to either recharge the deeper regional aquifer from the supra-permafrost system or facilitating discharge from the deeper aquifer (Bense et al., 2011; Walvoord et al., 2012; Lamontagne-Hallé et al., 2018). An easier flow of groundwater may increase the discharge of groundwater into the rivers (Ge et al., 2011; Walvoord et al., 2012; Lamontagne-Hallé, 2018). However, this phenomenon only holds if sufficient water is available to replenish the increased discharge. Otherwise, there will be an overall lowering of the water table in the recharge portion of the catchment (Ge et al., 2011). Michelle et al. (2016) suggest that permafrost loss is more likely to contribute to baseflow increases in discontinuous permafrost than in continuous permafrost, with permafrost tending to be cold and thick. The results of this study have shown that baseflow is increasing in the zone of continuous permafrost as well.
5.6.2 Glaciers, rock glaciers, and aufeis
Another possible contribution to streamflow increase could be the meltwater from glaciers, at least in the Indigirka River basin where glaciers take up to 0.12 % of the total basin area. Dyurgerov and Carter (2004) studied glacier retreat and their input into increased streamflow in Arctic rivers. Bliss et al. (2014) assessed the global-scale response of glacier runoff to climate change based on scenario modeling and have proposed that the Canadian and Russian Arctic region exhibits steady increases in glacier runoff consequently changing the hydrological regime.
Under the current climate, the glaciers of the region melt more intensively in July and August, so their input to streamflow would be expected during those months. In the headwaters of the Indigirka River (ID 3488, 51 100 km2) the area occupied by glaciers is the highest among the studied rivers and amounts to 0.30 % of the basin area; however, no significant trend of streamflow is observed in July and August. The next downstream gauge of the Indigirka River (ID 3489, 83 500 km2) exhibits a monotonic increase in streamflow in August from 1944 to 2015, which is mainly explained by increasing streamflow of its tributary, the Elgi River (ID 3507), where no glaciers are found. Huss and Hock (2018) estimated the hydrological response to current and future glacier mass loss; according to their simulations, the annual maximum input of glacier runoff to streamflow in the Indigirka River basin was passed in the period 1980–2010 and is expected to decline in the future. It will also be accompanied by a change in the timing of glacier runoff. In the Indigirka River basin, glacier runoff is expected to increase by 20 %–40 % in June and drop by 20 % on average in other months (Huss and Hock, 2018).
Liljedahl et al. (2017) have found that in Jarvis Creek (634 km2), a sub-basin of the Tanana and Yukon rivers, the excess discharge sourced from mountain glaciers has not only increased headwater streamflow, aquifer recharge, and storage but has also increased aquifer capacity – all with the final effect of increasing winter discharge in lower gauges. Ananicheva (2014) estimated the loss of glacier ice volumes for the Suntar–Khayata and Chersky ranges as 1.7 and 1.4 km3 for the periods 1945–2003 and 1970–2003, respectively; 1.7 km3 in 58 years would give an additional inflow of 0.35 mm yr−1, which is comparable with the increase in streamflow in the Indigirka River (ID 3489) in November and December for the same period. The fact that the change points of the increase in streamflow occur in late autumn – early winter occurred about 10–18 years earlier at a downstream gauge (ID 3489) in comparison with a headwater gauge (ID 3488) – does not contradict this hypothesis. But the same pattern of more early change points at a downstream gauge is observed at nested gauges of the Adycha River (ID 3443, 3445) where glacier impact would be negligible due to the tiny area in this catchment (about 1.35 km2 in total).
The melt of rock glaciers and aufeis, which is widespread in the study area, may have a similar effect of additional water input. According to Lytkin and Galanin (2016), 540 rock glaciers are identified within the Suntar–Khayata Range with a distribution density of 8.4 objects per 100 km2.
The assessment of aufeis area conducted for the Indigirka River basin based on recent Landsat imagery analysis has shown that the number of aufeis fields increased by 35 %, but the total area decreased by 1.6 times (Makarieva et al., 2019a) in comparison with the data by Simakov and Shilnikovskaya (1958). The current aufeis area share for the studied watersheds in the Indigirka River basin ranges from 0.26 % to 0.80 % (Makarieva et al., 2019a), though historical estimations are higher. For example, Tolstikhin (1974) estimates that relative aufeis area averages 0.4 % to 1.3 %, reaching 4 % in the basins of some rivers. Due to changed conditions of aufeis functioning in the current warmer climate, they may contribute to the observed increase in streamflow in May and June at several studied gauges due to more active melting.
5.6.3 Geotectonic conditions
A significant positive trend of annual streamflow was identified only for four gauges. Arzhakova (2001) emphasizes that the rivers Elgi (ID 3507, the Indigirka River basin) and Adycha (IDs 3443 and 3445, the Yana River basin) cross tectonic dislocation zones. The area is characterized by modern seismotectonic activities (Imaeva et al., 2017). Taliks, which form within faults and excessive jointing zones, supply rivers even in extreme winter conditions (Romanovsky, 1983). Glotov et al. (2011) proposed that in particular years during sublittoral seismic activity periods, winter streamflow fluctuations in the Kolyma River may depend on geotectonic extensions and compressions that occur in the region via riverbed through-taliks. Savelieva et al. (2000) pointed to redistribution of the water budget between the upper (thaw layer) water and groundwater beneath the permafrost. According to Savelieva et al. (2000), the increases in temperature and thickness of the permafrost active layer may enhance the water inputs from the seasonal thaw layer into the groundwaters beneath the permafrost through tectonic trenches and the lake's talik zone.
5.7 Overall impacts to the ecosystem
This study has identified increases in runoff in rivers discharging to the Arctic Ocean. Increasing volumes of freshwater flows to the Arctic Ocean could lead to a significant weakening of the thermohaline circulation, changing sea stratification, and sea ice formation (Arnell, 2005; Lique et al., 2016, Weatherly and Walsh, 1996). The total volume of water being discharged from the Yana and Indigirka rivers is around 84 km3 (about 1.7 % of total freshwater discharge to the Arctic). As a result, changes in these two basins are unlikely to impact the Arctic by themselves, but even subtle changes in river streamflow may have large implications for the ocean–climate system (Miller and Russel, 2000). However, if the changes in hydroclimate seen in these basins are being felt across other Arctic basins, impacts on the Arctic Ocean could be significant.
Approximately 85 % of the total terrestrial runoff to the Arctic Ocean is supplied by rivers draining from Russia (Aagaard and Carmack, 1989). Moreover, Makarieva et al. (2019b) have shown that most runoff of the Indigirka River is generated in mountainous areas and not from the coastal plains. Therefore, the analysis of this database, including the mountainous upstreams of the Yana and Indigirka rivers, is particularly timely.
Forman and Johnson (1996) have previously identified the assessment of volume, timing, and natural variability of Russian river discharge as a major priority in Arctic science. In particular, the Indigirka is one of the two largest rivers flowing into the East Siberian Sea (Anderson et al., 2011), and thus changes in flows from the Indigirka River have the potential to impact acidity, nutrients, and carbon cycling in this sea (Semiletov et al., 2016).
In terms of ecological impacts, increases in discharge, velocity, temperature, and concentration of suspended materials exert important effects on primary production and food-web dynamics in rivers and estuaries (Scrimgeour et al., 1994). Flooding associated with increased discharge is a primary control on the exchange of sediment and organic carbon between rivers and Arctic floodplains (Smith and Alsdorf, 1998).
Analysis of data from the Yana and Indigirka River basins has shown increases in annual air temperature for the region of around 2.0 ∘C over the last 50 years (1966–2015). Much of this increase has occurred in the late autumn and spring. Precipitation has shown a decrease in the late winter (February–March) and exhibits little change in other seasons. However, the increases in air temperature have led to a shift in the rain–snow ratio, with more rain at the expense of snow in May and September in mountainous areas. In total, snow loss can be estimated at around 20–25 mm, half of which now falls as rain and the other half as a decrease in late winter precipitation. The increase in air temperature is also reflected in soil temperature and the length of the thaw season.
Despite the slight decrease in overall precipitation, analysis of daily discharge data for 22 gauging stations in the Yana and Indigirka River basins (1936–2015) has revealed, at most of the stations, statistically significant (p<0.05) positive trends in monthly streamflow during the autumn–winter period (September through December) and in the spring flood (May–June). Changes in spring flow are also seen through the spring freshet occurring 4 to 10 d earlier over the period of record. Total annual flow has increased significantly in the upstream tributaries of the Yana and Indigirka rivers.
Most of the increases in streamflow are seen via break points, rather than showing a monotonic increase over the entire period of record. The structure of the timing of these changes is very complex; however, in general, the changes occur in the Yana River basin 10 years before the Indigirka River basin. Changes in winter streamflow are seen in the larger river basins (lower-elevation gauges) before they are seen in the smaller river basins. Analysis of monthly data is required in order to identify these changes, as in many cases, changes in annual flow are not significant. Additionally, analysis of other datasets such as monthly precipitation and temperature is required in order to develop hypotheses regarding the potential causes of these changes in streamflow.
Increases in streamflow in the autumn–winter period are likely mainly caused by a shift in precipitation from snow to rain in September, with consequent increases in streamflow in that month, continuing into October and later months. Decreased depth of river ice and a better connection of the surface and groundwater due to the deepening of the active layer and prolonging the freeze-free period may also be causing higher winter flow.
There are no changes seen in spring discharge in the last 50 years (from around 1966) except at several upstream, mountainous gauges in both studied river basins. Curiously, snow losses and the earlier timing of the spring freshet in May do not lead to a decrease in streamflow in June. This additional input of water is likely related to the regional warming trend impacting more intensive melting of the cryosphere elements such as permafrost, glaciers, and aufeis.
It is difficult to directly attribute changes in streamflow to definite causes, and it is almost certainly a consequence of a complicated interplay between different changing water storages and pathways in warming permafrost. The possibility also exists of additional water input via precipitation higher in the mountains, but this hypothesis cannot be verified with current observational data. The observed changes agree with reported positive trends in Arctic river discharge, albeit with low confidence (IPCC, 2014), and the importance of local factors in streamflow response to climate change over Siberia (AMAP, 2017). Increased streamflow may also cause changes to the Arctic Ocean through an increased freshwater flux and decreased thermohaline circulation.
Combined monthly hydrometeorological data used in this study are publicly available and can be downloaded from https://doi.org/10.1594/PANGAEA.892775 (Makarieva et al., 2018b).
The supplement related to this article is available online at: https://doi.org/10.5194/tc-13-1635-2019-supplement.
All the authors discussed the results presented in this paper. OM, NN, and LL performed the analysis of hydrological and meteorological data. AS provided and analyzed soil data. The paper was written by OM, NN, and DAP with relevant comments from all the coauthors. The figures were designed by NN in close collaboration with OM and DAP.
The authors declare that they have no conflict of interest.
The authors are grateful to two anonymous reviewers and the handling editor, Valentina Radic, whose comments and suggestions made the paper considerably better.
The study was partially funded by RFBR under research project no. 18-45-140065.
This paper was edited by Valentina Radic and reviewed by two anonymous referees.
Aagaard, K. and Carmack, E. C.: The role of sea ice and other fresh water in the arctic circulation, J. Geophys. Res., 94, 14485–14498, 1989.
AMAP: Snow, Water, Ice and Permafrost. Summary for Policy-makers, Arctic Monitoring and Assessment Programme (AMAP), Oslo, Norway, 20 pp., 2017.
Ananicheva, M. D.: Estimation of the areas, volumes and heights of the boundary of the feeding of glacier systems of the Northeast of Russia from the space images of the beginning of the 21st century, Ice and Snow, 1, 35–48, 2014.
Anderson, L. G., Björk, G., Jutterström, S., Pipko, I., Shakhova, N., Semiletov, I., and Wåhlström, I.: East Siberian Sea, an Arctic region of very high biogeochemical activity, Biogeosciences, 8, 1745–1754, https://doi.org/10.5194/bg-8-1745-2011, 2011.
Arnell, N. W.: Implications of climate change for freshwater inflows to the Arctic Ocean, J. Geophys. Res., 110, D07105, https://doi.org/10.1029/2004JD005348, 2005.
Arzhakova, S. K.: The winter flow of the rivers of the permafrost zone of Russia. Gidrometeoizdat, St. Petersburg, 2001 (in Russian).
Bense, V. F., Kooi, H., Ferguson, G., and Read, T.: Permafrost degradation as a control on hydrogeological regime shifts in a warming climate, J. Geophys. Res., 117, F03036, https://doi.org/10.1029/2011JF002143, 2011.
Berezovskaya, S., Yang, D., and Kane, D. L.: Compatibility analysis of precipitation and runoff trends over the large Siberian watersheds, Geophys. Res. Lett., 31, L21502, https://doi.org/10.1029/2004GL021277, 2004.
Berghuijs, W. R., Woods, R. A., and Hrachowitz, M.: A precipitation shift from snow towards rain leads to a decrease in streamflow, Nat. Clim. Change, 4, 583–586, 2014.
Bliss, A., Hock, R., and Radić, V.: Global response of glacier runoff to twenty-first century climate change, J. Geophys. Res.-Earth, 119, 717–730, https://doi.org/10.1002/2013JF002931, 2014.
Bring, A. and Destouni, G.: Arctic climate and water change: Model and observation relevance for assessment and adaptation, Surv. Geophys., 35, 853–877, 2014.
Bring, A., Fedorova, I., Dibike, Y., Hinzman, L., Mård, J., Mernild, S. H., Prowse, T., Semenova, O., Stuefer, S. L., and Woo, M.-K.: Arctic terrestrial hydrology: A synthesis of processes, regional effects, and research challenges, J. Geophys. Res.-Biogeo., 121, 621–649, https://doi.org/10.1002/2015JG003131, 2016.
Brown, J., Ferrians Jr., O. J., Heginbottom, J. A., and Melnikov, E.S. (Eds.): Circum-Arctic map of permafrost and ground-ice conditions, U.S. Geological Survey in Cooperation with the Circum-Pacific Council for Energy and Mineral Resources, Washington, DC, Circum-Pacific Map Series CP-45, scale , 1 sheet, https://doi.org/10.3133/cp45, 1997.
Buishand, T. A.: Some methods for testing the homogeneity of rainfall records, J. Hydrol., 58, 11–27, 1982.
Callaghan, T. V., Johansson, M., Prowse, T. D., Olsen, M. S., and Reiersen, L.-O.: Arctic Cryosphere: Changes and Impacts, Ambio, 40, 3–5, https://doi.org/10.1007/s13280-011-0210-0, 2011.
Copernicus Climate Change Service: Project #C3S_422_LOT1_SMHI, Swedish Meteorological and Hydrological Institute, 2017–2019.
Dyurgerov, M. B. and Carter, C. L.: Observational evidence of increases in freshwater inflow to the Arctic Ocean, Arct. Antarct. Alp. Res., 36, 117–122, https://doi.org/10.1657/1523-0430(2004)036, 2004.
Jamalov, R. G., Krichevets, G. N., and Safronova, T. I.: Modern changes of water resources in the Lena River basin, Water Resour., 39, 131–145, 2012.
Explanatory note to the geocryological map of the USSR, scale 1:2 500 000, edited by: Kondratieva, K. A., Dunaeva, E. N., Trush, N. A., Gavrilov, A. V., Afanasenko, V. E., Khrutsky, S. F., and Ershov, E. D., Ministry of Geology of the USSR, 125 pp., 1991 (in Russian).
Fedorov, A. N., Ivanova, R. N., Perk, H., Hiyama, T., and Iijima, Y.: Recent air temperature changes in the permafrost landscapes of northeastern Eurasia, Polar Sci., 8, 114–128, https://doi.org/10.1016/j.polar.2014.02.001, 2014.
Fedorov, A. N., Vasilyev, N. F., Torgovkin, Y. I., Shestakova, A. A., Varlamov, S. P., Zheleznyak, M. N., Shepelev, V. V., Konstantinov, P. Y., Kalinicheva, S. S., Basharin, N. I., Makarov, V. S., Ugarov, I. S., Efremov, P. V., Argunov, R. N., Egorova, L. S., Samsonova, V. V., Shepelev, A. G., Vasiliev, A. I., Ivanova, R. N., Galanin, A. A., Lytkin, V. M., Kuzmin, G. P., and Kunitsky, V. V.: Permafrost-Landscape Map of the Republic of Sakha (Yakutia) on a Scale , Geosciences, 8, 465, https://doi.org/10.3390/geosciences8120465, 2018.
Forman, S. L. and Johnson, J. L.: Reports of National Science Foundation Arctic System Science sponsored workshops to define research priorities for Eurasian Arctic land-shelf systems, Byrd Polar Research Center, Columbus, Ohio State University, Miscellaneous Series M-397, 51 pp, 1996.
Frey, K. E. and McClelland, J. W.: Impacts of permafrost degradation on arctic river biogeochemistry, Hydrol. Process., 23, 169–182, 2009.
Ge, S., McKenzie, J., Voss, C., and Wu, Q.: Exchange of groundwater and surface-water mediated by permafrost response to seasonal and long term air temperature variation, Geophys. Res. Lett., 38, L14402, https://doi.org/10.1029/2011GL047911, 2011.
Georgievsky, M.: Water resources of the Russian rivers and their changes, Proc. IAHS, 374, 75–77, https://doi.org/10.5194/piahs-374-75-2016, 2016.
GLIMS and NSIDC: Global Land Ice Measurements from Space glacier database, Compiled and made available by the international GLIMS community and the National Snow and Ice Data Center, Boulder CO, USA, https://doi.org/10.7265/N5V98602, 2005 (updated 2017).
Glotov, V. E., Glotova, L. P., and Ushakov, M. V.: Abnormal changes in the regime of the water flow of the Kolyma River in the winter low water. Cryosphere of the Earth. XV, 1, 52-60, 2011.
Glotova, L. P. and Glotov, V. E.: General regularities of underground feeding of rivers in the North-East Russia, News of Samara Scientific Center of the Russian Academy of Sciences, 17, 63–69, 2015 (in Russian).
Günther, F., Overduin, P. P., Yakshina, I. A., Opel, T., Baranskaya, A. V., and Grigoriev, M. N.: Observing Muostakh disappear: permafrost thaw subsidence and erosion of a ground-ice-rich island in response to arctic summer warming and sea ice reduction, The Cryosphere, 9, 151–178, https://doi.org/10.5194/tc-9-151-2015, 2015.
Grave, N., Gavrilova, M., Gravis, G., Katasonov, E., Klyukin, N., Koreysha, G., Kornilov, B., and Chistotinov, L.: The freezing of the earth's surface and glaciation on the ridge Suntar-Khayata (Eastern Yakutia), Nauka, Moscow, 1964 (in Russian).
Gurevich, E. V.: Influence of air temperature on the river runoff in winter (the Aldan river catchment case study), Russ. Meteorol. Hydrol., 34, 628–633, 2009 (in Russian).
Hinzman, L. D., Deal, C. J., McGuire, A. D., Mernild, S. H., Polyakov, I. V., and Walsh, J. E.: Trajectory of the Arctic as an integrated system, Ecol. Appl., 23, 1837–1868, https://doi.org/10.1890/11-1498.1, 2013.
Holland, M. M., Finnis, J., Barrett, A. P., and Serreze, M. C.: Projected changes in Arctic Ocean freshwater budgets, J. Geophys. Res., 112, G04S55, https://doi.org/10.1029/2006JG000354, 2007.
Holmes, R. M., Coe, M. T., Fiske, G. J., Gurtovaya, T., McClelland, J. W., Shiklomanov, A. I., Spencer, R. G. M., Tank, S. E., and Zhulidov, A. V.: Climate change impacts on the hydrology and biogeochemistry of Arctic rivers, in: Global impacts of climate change on inland waters: Impacts and mitigation for ecosystems and societies, edited by: Goldman, C. R., Kumagai, M., and Robarts, R. D., WileyBlackwell, Hoboken, New Jersey, 3–26, 2013.
Huntington, T. G.: Climate change, growing season length, and transpiration: Plant response could alter hydrologic regime, Plant Biol., 6, 651–653, 2004.
Huss, M. and Hock, R.: Global-scale hydrological response to future glacier mass loss, Nat. Clim. Change, 8, 135–140, https://doi.org/10.1038/s41558-017-0049-x, 2018.
Imaeva, L. P., Gusev, G. S., Imaev, V. S., Ashurkov, S. V., Melnikova, V. I., and Seredkina, A. I.: Geodynamic activity of advanced structures and tectonic stress fields of northeast Asia, Geodynamics and Tectonophysics, 8, 737-–768, https://doi.org/10.5800/GT-2017-8-4-0315, 2017 (in Russian).
IPCC: Climate Change 2014: Synthesis Report, Contribution of Working Groups I, II and III to the Fifth Assessment Report of the Intergovernmental Panel on Climate Change, IPCC, Geneva, Switzerland, 151 pp., 2014.
Imaeva, L. P., Gusev, G. S., Imaev, V. S., Ashurkov, S. V., Melnikova, V. I., and Seredkina, A. I.: Geodynamic activity of advanced structures and tectonic stress fields of northeast Asia, Geodynamics and Tectonophysics, 8, 737-–768, https://doi.org/10.5800/GT-2017-8-4-0315, 2017 (in Russian).
Ivanova, R. N.: Extremely low air temperatures in Eurasia, Vestnik YSU, 3, 13–19, 2006 (in Russian).
Jamalov, R. G., Krichevets, G. N., and Safronova, T. I.: Modern changes of water resources in the Lena River basin, Water Resour., 39, 131–145, 2012.
Jepsen, S. M., Voss, C. I., Walvoord, M. A., Minsley, B. J., and Rover, J.: Linkages between lake shrinkage/expansion and sublacustrine permafrost distribution determined from remote sensing of interior Alaska, USA, Geophys. Res. Lett., 40, 882–887, https://doi.org/10.1002/grl.50187, 2013.
Karlsson, J. M., Jaramillo, F., and Destouni, G.: Hydro-climatic and lake change patterns in Arctic permafrost and non-permafrost areas, J. Hydrol., 529, 134–145, https://doi.org/10.1016/j.jhydrol.2015.07.005, 2015.
Kendall, M. G.: Rank Correlation Methods, Griffin, London, UK, 1975.
Kirillina, K. S.: Current trends of climate change of the republic of Sakha (Yakutia), Scientific memories of the Russian State Hydrometeorological University, Russian State Hydrometeorological University, St. Petersburg, ISSN: 2074-2762, 2013 (in Russian).
Kundzewicz, Z. W. and Robson, A. J.: Change detection in hydrological records – a review of the methodology, Hydrolog. Sci. J., 49, 7–19, 2004.
Lamontagne-Hallé, P., McKenzie, J. M., Kurylyk, B. L., and Zipper, S. C.: Changing groundwater discharge dynamics in permafrost regions, Environ. Res. Lett., 13, 084017, https://doi.org/10.1088/1748-9326/aad404, 2018.
Lamoureux, S. F. and Lafrenière, M. J.: More than just snowmelt: integrated watershed science for changing climate and permafrost at the Cape Bounty Arctic Watershed Observatory, WIREs Water, 5, e1255, https://doi.org/10.1002/wat2.1255, 2018.
Lebedeva, L.S., Makarieva, O.M., and Vinogradova, T.A.: Spatial variability of the water balance elements in mountain catchments in the North-East Russia (case study of the Kolyma Water Balance Station). Meteorology and Hydrology J., 4, 90-101, 2017 (in Russian).
Lehmann, E. L.: Nonparametrics, Statistical methods based on ranks, Holden-Day, Inc., California, USA, 1975.
Liljedahl, A. K., Gädeke, A., O'Neel, S., Gatesman, T. A., and Douglas, T. A.: Glacierized headwater streams as aquifer recharge corridors, subarctic Alaska, Geophys. Res. Lett., 44, 6876–6885, https://doi.org/10.1002/2017GL073834, 2017.
Lesack, L. F. W., Marsh, P., Hicks, F. E., and Forbes, D. L.: Timing, duration, and magnitude of peak annual water-levels during ice breakup in the Mackenzie Delta and the role of river discharge, Water Resour. Res., 49, 8234–8249, https://doi.org/10.1002/2012WR013198, 2013.
Lique, C., Holland, M. M., Dibike, Y. B., Lawrence, D. M., and Screen, J. A.: Modeling the Arctic freshwater system and its integration in the global system: Lessons learned and future challenges, J. Geophys. Res.-Biogeo., 121, 540–566, https://doi.org/10.1002/2015JG003120, 2016.
Lytkin, V. M. and Galanin, A. A.: Rock glaciers in the Suntar-Khayata Range, Ice and Snow, 56, 511–524, https://doi.org/10.15356/2076-6734-2016-4-511-524, 2016 (in Russian).
Magritsky, D. V., Mikhailov, V. N., Korotaev, V. N., and Babich, D. B.: Changes in hydrological regime and morphology of river deltas in the Russian Arctic, in: Proceedingsof HP1, IAHS-IAPSO-IASPEI Assembly, Gothenburg, Sweden, July 2013, IAHS Publ. 358, 67–79, 2013.
Majhi, I. and Yang, D.: Cold Region Hydrology in a Changing Climate, IAHS Publ. 346, 39–43, 2011.
Makarieva, O., Nesterova, N., Lebedeva, L., and Sushansky, S.: Water balance and hydrology research in a mountainous permafrost watershed in upland streams of the Kolyma River, Russia: a database from the Kolyma Water-Balance Station, 1948–1997, Earth Syst. Sci. Data, 10, 689–710, https://doi.org/10.5194/essd-10-689-2018, 2018a.
Makarieva, O., Nesterova, N., and Sherstyukov, A.: Monthly hydro-climate database for the Yana and Indigirka Rivers basins, Northern Eurasia, PANGAEA, https://doi.org/10.1594/PANGAEA.892775, 2018b.
Makarieva, O., Shikhov, A., Nesterova, N., and Ostashov, A.: Historical and recent aufeis in the Indigirka River basin (Russia), Earth Syst. Sci. Data, 11, 409–420, https://doi.org/10.5194/essd-11-409-2019, 2019a.
Makarieva, O., Nesterova, N., Lebedeva, L., and Vinogradova, T.: Modeling runoff formation processes in the high-mountain permafrost zone of Eastern Siberia (a case study of the Suntar-Khayata Range), Geography and Natural Resources, 1, 178–186, https://doi.org/10.21782/GiPR0206-1619-2019-1(178-186), 2019b. (in Russian)
Mann, H. B.: Nonparametric tests against trend, Econometrica, 13, 245–259, 1945.
McClelland, J. W., Holmes, R. M., Peterson, B. J., and Stieglitz, M.: Increasing river discharge in the Eurasian Arctic: Consideration of dams, permafrost thaw, and fires as potential agents of change, J. Geophys. Res., 109, D18102, https://doi.org/10.1029/2004JD004583, 2004.
McClelland, J. W., Déry, S. J., Peterson, B. J., Holmes, R. M., and Wood, E. F.: A pan-arctic evaluation of changes in river discharge during the latter half of the 20th century, Geophys. Res. Lett., 33, L06715, https://doi.org/10.1029/2006gl025753, 2006.
Mikhailov, V. M.: Floodplain taliks of North-East of Russia, Novosibirsk. Geo., 244 pp., 2013 (in Russian).
Miller, J. R. and Russell, G. L.: Projected impact of climate change on the freshwater and salt budgets of the Arctic Ocean by a glob al climate mode l, Geophys. Res. Lett., 27, 1183–1186, https://doi.org/10.1029/1999GL007001, 2000.
Milliman, J. D., Farnsworth, K. L., Jones, P. D., Xu, K. H., and Smith, L. C.: Climatic and anthropogenic factors affecting river discharge to the global ocean, 1951–2000, Global Planet. Change, 62, 187–194, https://doi.org/10.1016/j.gloplacha.2008.03.001, 2008.
Muskett, R. R. and Romanovsky, V. E.: Groundwater storage changes in arctic permafrost watersheds from GRACE and in situ measurements, Environ. Res. Lett., 4, 045009, https://doi.org/10.1088/1748-9326/4/4/045009, 2009.
Pavlov, A. V. and Malkova, G. V.: Small-scale mapping of trends of the contemporary ground temperature changes in the Russian North, Earth's Cryosphere, 13, 32–39, 2009.
Peterson, B. J., Holmes, R. M., McClelland, J. W., Vörösmarty, C. J., Lammers, R. B., Shiklomanov, A. I., Shiklomanov, I. A., and Rahmstorf C.: Increasing river discharge to the Arctic Ocean, Science, 298, 2171–2173, https://doi.org/10.1126/science.1077445, 2002.
Peterson, B. J., McClelland, J., Curry, R., Holmes, R. M., Walsh, J. E., and Aagaard, K.: Trajectory shifts in the Arctic and Subarctic freshwater cycle, Science, 313, 1061–1066, 2006.
Pettitt, A. N.: A non-parametric approach to the change-point problem, J. R. Stat. Soc. C-Appl., 28, 126–135, 1979.
Rawlins, M. A., Steele, M., Holland, M. M., Adam, J. C., Cherry, J. E., Francis, J. A., Groisman, P. Y., Hinzman, L. D., Huntington, T. G., Kane, D. L., Kimball, J. S., Kwok, R., Lammers, R. B., Lee, C. M., Lettenmaier, D. P., McDonald, K. C., Podest, E., Pundsack, J. W., Rudels, B., Serreze, M. C., Shiklomanov, A., Skagseth, Ø., Troy, T. J., Vörösmarty, C. J., Wensnahan, M., Wood, E. F., Woodgate, R., Yang, D., Zhang, K., and Zhang, T.: Analysis of the Arctic System for Freshwater Cycle Intensification: Observations and Expectations, J. Climate, 23, 5715–5737, https://doi.org/10.1175/2010JCLI3421.1, 2010.
Reedyk, S., Woo, M. K., and Prowse, T. D.: Contribution of icing ablation to flow in a discontinuous permafrost area, Can. J. Earth Sci., 32, 13–20, 1995.
Rennermalm, A. K., Wood, E. F., and Troy, T. J.: Observed changes in pan-arctic cold-season minimum monthly river discharge, Clim. Dynam., 35, 923–939, 2010.
Romanovsky, N. N.: Underground waters of cryolithozone, Moscow State University, Moscow, 1983 (in Russian).
Romanovsky, V. E., Sazonova, T. S., Balobaev, V. T., Shender, N. I., and Sergueev, D. O.: Past and recent changes in air and permafrost temperatures in eastern Siberia, Global Planet. Change, 56, 399–413, https://doi.org/10.1016/j.gloplacha.2006.07.022, 2007.
Romanovsky, V. E., Smith, S. L., and Christiansen, H. H.: Permafrost thermal state in the polar Northern Hemisphere during the International Polar Year 2007–2009: A synthesis, Permafrost Periglac., 21, 106–116, https://doi.org/10.1002/ppp.689, 2010.
Rood, S. B., Kaluthota, S., Philipsen, L. J., Rood, N. J., and Zanewich, K. P.: Increasing discharge from the Mackenzie River system to the Arctic Ocean, Hydrol. Process., 31, 150–160, https://doi.org/10.1002/hyp.10986, 2017.
Savelieva, N. I., Semiletov, I. P., Vasilevskaya, L. N., and Pugach, S. P.: A climate shift in seasonal values of meteorological and hydrological parameters for Northeastern Asia, Prog. Oceanogr., 47, 279–297, 2000.
Scrimgeour, G. J., Prowse, T. D., Culp, J. M., and Chambers, P. A.: Ecological effects of river ice break-up: a review and perspective, Freshwater Biol., 32, 261–275, 1994.
Semiletov, I., Pipko, I., Gustafsson, O., Anderson, L. G., Sergienko, V., Pugach, S., Dudarev, O., Charkin, A., Gukov, A., Bröder, L., Andersson, A., Spivak, E., and Shakhova, N.: Acidification of East Siberian Arctic Shelf waters through addition of freshwater and terrestrial carbon, Nat. Geosci., 9, 361–365, 2016.
Sen, P. K.: Estimates of the regression coefficient based on Kendall's tau, J. Am. Statist. Assoc., 63, 1379–1389, 1968.
Serreze, M. C., Bromwich, D. H., Clark, M. P., Etringer, A. J., Zhang, T., and Lammers, R.: The large-scale hydroclimatology of the terrestrial Arctic drainage, J. Geophys. Res., 108, 8160, https://doi.org/10.1029/2001JD000919, 2003.
Shepelev, V. V.: Suprapermafrost waters in the cryolithozone, Novosibirsk. Geo., 169 pp., 2011 (in Russian).
Sherstyukov, A. B.: Correlation of soil temperature with air temperature and snow cover depth in Russia, Earth's Cryosphere, XII, 79–87, 2008.
Sherstyukov, A. B. and Sherstyukov, B. G.: Spatial features and new trends in thermal conditions of soil and depth of its seasonal thawing in the permafrost zone, Russ. Meteorol. Hydrol., 40, 73–78, https://doi.org/10.3103/S1068373915020016, 2015.
Shiklomanov, A. I. and Lammers, R. B.: Changing Discharge Patterns of High-Latitude Rivers, in: Climate Vulnerability: Understanding and Addressing Threats to Essential Resources, 5, 161–175, https://doi.org/10.1016/B978-0-12-384703-4.00526-8, 2013.
Shiklomanov, A. I. and Lammers, R. B.: River ice responses to a warming Arctic – recent evidence from Russian rivers, Environ. Res. Lett., 9, 035008, https://doi.org/10.1088/1748-9326/9/3/035008, 2014.
Shiklomanov, A. I., Yakovleva, T. I., Lammers, R. B., Karasev, I. Ph., Vörösmarty, C. J., and Linder, E.: Cold region river discharge uncertainty – estimates from large Russian rivers, J. Hydrol., 326, 231–56, https://doi.org/10.1016/j.jhydrol.2005.10.037, 2006.
Shiklomanov, A. I., Lammers, R. B., Rawlins, M. A., Smith, L. C., and Pavelsky, T. M.: Temporal and spatial variations in maximum river discharge from a new Russian dataset, J. Geophys. Res., 112, G04S53, https://doi.org/10.1029/2006JG000352, 2007.
Shkolnik, I., Pavlova, T., Efimov, S., and Zhuravlev, S.: Future changes in peak river flows across northern Eurasia as inferred from an ensemble of regional climate projections under the IPCC RCP8.5 scenario, Clim. Dynam., 50, 215–230, https://doi.org/10.1007/s00382-017-3600-6, 2017.
Simakov, A. S. and Shilnikovskaya, Z. G.: The map of the naleds of the North-East of the USSR. A Brief Explanatory Note, The North-Eastern Geological Survey of the Main Directorate of Geology and Subsoil Protection, Magadan, 40 pp., 1958 (in Russian).
Smith, L. C.: Trends in Russian arctic river-ice formation and breakup, 1917 to 1994, Phys. Geogr., 21, 46–56, 2000.
Smith, L. C. and Alsdorf, D. E.: Control on sediment and organic carbon delivery to the Arctic Ocean revealed with space-borne synthetic aperture radar: Ob' River, Siberia, Geology, 26, 395–398, 1998.
Sokolov, B. L.: Aufeis (naleds) and river runoff, Leningrad, Gidrometeoizdat, 1975 (in Russian).
Spence, C., Kokelj, S. V., and Ehsanzadeh, E.: Precipitation trends contribute to streamflow regime shifts in northern Canada, Cold Region Hydrology in a Changing Climate, IAHS publication 346, 3–8, 2011.
State water cadastre: Main hydrological characteristics (for 1971–1975 and the whole period of observation), Volume 17, Leno-Indigirsky district, Leningrad, Gidrometeoizdat, 1979 (in Russian).
St. Jacques, J.-M., and Sauchyn, D. J.: Increasing winter baseflow and mean annual streamflow from possible permafrost thawing in the northwest territories, Canada, Geophys. Res. Lett., 36, 329–342, 2009.
Stieglitz, M., Déry, S. J., Romanovsky, V. E., and Osterkamp, T. E.: The role of snow cover in the warming of arctic permafrost, Geophys. Res. Lett., 30, 1721, https://doi.org/10.1029/2003GL017337, 2003.
Streletsky, D. A., Sherstiukov, A. B., Frauenfeld, O. W., and Nelson, F. E.: Changes in the 1963–2013 shallow ground thermal regime in Russian permafrost regions, Environ. Res. Lett., 10, 125005, https://doi.org/10.1088/1748-9326/10/12/125005, 2015.
Stuefer, S. L., Arp, C. D., Kane, D. L., and Liljedahl, A. K.: Recent Extreme Runoff Observations From Coastal Arctic Watersheds in Alaska, Water Resour. Res., 53, 9145–9163, https://doi.org/10.1002/2017WR020567, 2017.
Tan, A., Adam, J. C., and Lettenmaier, D. P.: Change in spring snowmelt timing in eurasian arctic rivers, J. Geophys. Res., 116, D03101, https://doi.org/10.1029/2010JD014337, 2001.
Tananaev, N. I., Makarieva, O. M., and Lebedeva, L. S.: Trends in annual and extreme flows in the Lena River basin, Northern Eurasia, https://doi.org/10.1002/2016GL070796, 2016.
Tolstikhin, O. N.: Aufeis and underground waters in the Northeast USSR, Novosibirsk, Nauka, 1974 (in Russian).
Vasiliev, I. S. and Torgovkin, I. I.: Spatial distribution of precipitation in Yakutia, Meteorology and Hydrology, 6, 23–32, 2002.
Velicogna, I., Tong, J., Zhang, T., and Kimball, J. S.: Increasing Subsurface Water Storage in Discontinuous Permafrost Areas of the Lena River Basin, Eurasia, Detected From GRACE, Geophys. Res. Lett., 39, L09403, https://doi.org/10.1029/2012GL051623, 2012.
Walwoord, M. A. and Kurylyk, B.L.: Hydrologic impacts of thawing permafrost – A review, Vadose Zone J., 15, 20 pp., https://doi.org/10.2136/vzj2016.01.0010, 2016.
Walvoord, M. A., Voss, C. I., and Wellman, T. P.: Influence of permafrost distribution on groundwater flow in the context of climate-driven permafrost thaw: Example from Yukon Flats Basin, Alaska, United States, Water Resour. Res., 48, W07524, https://doi.org/10.1029/2011WR011595, 2012.
Weatherly, J. W. and Walsh, J. E.: The effects of precipitation and river runoff in a coupled ice-ocean model of the Arctic, Clim. Dynam., 12, 785–798, 1996.
Yang, D., Kane, D. L., Hinzman, L. D., Zhang, X., Zhang, T., and Hengchun Ye.: Siberian Lena River hydrologic regime and recent change, J. Geophys. Res., 107, 4694, https://doi.org/10.1029/2002JD002542, 2002.
Yang, D., Kane, D., Zhang, Z., Legates, D., and Goodison, B.: Bias corrections of long-term (1973–2004) daily precipitation data over the northern regions, Geophys. Res. Lett., 32, L19501, https://doi.org/10.1029/2005GL024057, 2005
Yang, D., Shi, X., and Marsh, P.: Variability and extreme of Mackenzie River daily discharge during 1973–2011, Quatern. Int., 380–381, 159–168, https://doi.org/10.1016/j.quaint.2014.09.023, 2015
Yoshikawa, K. and Hinzman, L. D.: Shrinking thermokarst ponds and groundwater dynamics in discontinuous permafrost near council, Alaska, Permafrost Periglac., 14, 151–160, 2003.
Yue, S., Pilon, P., and Cavadias, G.: Power of the Mann–Kendall and Spearman's rho tests for detecting monotonic trends in hydrological series, J. Hydrol., 259, 254–271, 2002
Zhizhin, V. I., Zheleznyak, M. N., and Pulyaev, N. A.: Cryogenic processes in the morphology formation of the mountain relief at the Suntar-Khayata Range, Bulletin of the Ammosova North-Eastern Federal University, 9, 73–79, 2012 (in Russian).