the Creative Commons Attribution 4.0 License.
the Creative Commons Attribution 4.0 License.
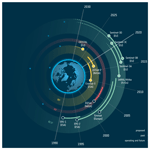
The Copernicus Polar Ice and Snow Topography Altimeter (CRISTAL) high-priority candidate mission
Michael Kern
Robert Cullen
Bruno Berruti
Jerome Bouffard
Tania Casal
Mark R. Drinkwater
Antonio Gabriele
Arnaud Lecuyot
Michael Ludwig
Rolv Midthassel
Ignacio Navas Traver
Tommaso Parrinello
Gerhard Ressler
Erik Andersson
Cristina Martin-Puig
Ole Andersen
Annett Bartsch
Sinead Farrell
Sara Fleury
Simon Gascoin
Amandine Guillot
Angelika Humbert
Eero Rinne
Andrew Shepherd
Michiel R. van den Broeke
John Yackel
The Copernicus Polar Ice and Snow Topography Altimeter (CRISTAL) mission is one of six high-priority candidate missions (HPCMs) under consideration by the European Commission to enlarge the Copernicus Space Component. Together, the high-priority candidate missions fill gaps in the measurement capability of the existing Copernicus Space Component to address emerging and urgent user requirements in relation to monitoring anthropogenic CO2 emissions, polar environments, and land surfaces. The ambition is to enlarge the Copernicus Space Component with the high-priority candidate missions in the mid-2020s to provide enhanced continuity of services in synergy with the next generation of the existing Copernicus Sentinel missions. CRISTAL will carry a dual-frequency synthetic-aperture radar altimeter as its primary payload for measuring surface height and a passive microwave radiometer to support atmospheric corrections and surface-type classification. The altimeter will have interferometric capabilities at Ku-band for improved ground resolution and a second (non-interferometric) Ka-band frequency to provide information on snow layer properties. This paper outlines the user consultations that have supported expansion of the Copernicus Space Component to include the high-priority candidate missions, describes the primary and secondary objectives of the CRISTAL mission, identifies the key contributions the CRISTAL mission will make, and presents a concept – as far as it is already defined – for the mission payload.
- Article
(1587 KB) - Full-text XML
- BibTeX
- EndNote
Earth's cryosphere plays a critical role in our planet's radiation and sea level budgets. Loss of Arctic sea ice is exacerbating planetary warming owing to the ice albedo feedback (e.g. Budyko, 1969; Serreze and Francis, 2006; Screen and Simmonds 2010), and loss of land ice is the principal source of global sea level rise (see Intergovernmental Panel on Climate Change, IPCC; SROCC, 2019). The rates and magnitudes of depletion of Earth's marine and terrestrial ice fields are among the most significant elements of future climate projections (Meredith et al., 2019). The Arctic provides fundamental ecosystem services (including fishery management and other resources), sustains numerous indigenous communities, and, due to sea ice loss, is emerging as a key area for economic exploitation. The fragile ecosystems are subject to pressures from a growing number of maritime and commercial activities. The potentially devastating contribution of the Antarctic ice sheet to global sea level rise is also subject to large uncertainties in ice mass loss, with high-end estimates of sea level contribution exceeding a metre of global mean sea level rise by 2100 (Edwards et al., 2019).
A long-term programme to monitor the Earth's polar ice, ocean and snow topography is important to stakeholders with interests in the Arctic and Antarctic. While Europe has a direct interest in the Arctic due to its proximity (see https://ec.europa.eu/environment/efe/news/integrated-eu-policy-arctic-2016-12-08_en, last access: 10 July 2020), the Arctic is also of interest to other countries and international communities. Changes in the Arctic environment affect strategic areas including politics, economics (e.g. exploitation of natural resources including minerals, oil and gas, and fish), and security. Besides economic impacts of Antarctic and Arctic changes (Whiteman et al., 2013), Europe's interest in both polar regions is due to their influence on patterns and variability in global climate change, thermohaline circulation, and the planetary energy balance. Last but not least, changes in the Arctic system have potential impacts on weather, with consequences for extreme events (Francis et al., 2017). The Copernicus Polar Ice and Snow Topography Altimeter (CRISTAL) mission described in this paper addresses the data and information requirements of these user communities with a particular focus on addressing Copernicus service requirements.
In the following section, we provide a background of the Copernicus programme and candidate missions that are being prepared by the European Space Agency (ESA) in partnership with the European Union (EU) in response to Copernicus user needs. In Sect. 3, we describe the objectives of the CRISTAL mission and its relation to the Copernicus services. We then discuss the key contributions from the CRISTAL mission in terms of both specific mission objectives and expected scientific contributions towards improved knowledge in Sect. 4. In Sect. 5, an overview of CRISTAL's current system concept and mode of operation is described. This section also highlights the use of heritage technology and needs driving technical advancements to improve observational capabilities beyond current missions. Conclusions and a current mission status statement are provided in Sect. 6.
Copernicus was established to fulfil the growing need amongst European policymakers to access accurate and timely information services to better manage the environment, understand and mitigate the effects of climate change, and ensure civil security. To ensure the operational provision of Earth observation data, the Copernicus Space Component (CSC) includes a series of seven space missions called “Copernicus Sentinels”, which are being developed by the ESA specifically for Global Monitoring for Environment and Security (GMES) and Copernicus. The Copernicus programme is coordinated and managed by the European Commission (EC). It includes Earth observation satellites, ground-based measurements, and services to process data to provide users with reliable and up-to-date information through a set of Copernicus services related to environmental and security issues.
The intense use of Copernicus has generated high expectations for an evolved Copernicus system. There is now a large set of defined needs and requirements. With respect to the polar regions, user and observation requirements have been identified, structured, and prioritized in a process led by the EC (Duchossois et al., 2018a, b). Two distinct sets of expectations have emerged from this user consultation process. Firstly, stability and continuity, while increasing the quantity and quality of Copernicus products and services, led to one set of requirements. They are distinctly addressed in the considerations for the next generation of the current Sentinel-1 to Sentinel-6 series (see e.g. European Commission, 2017). Emerging and urgent needs for new types of observations constitute a second distinct set of requirements that are mainly addressed through the evolution of the Copernicus Space Segment service. This evolution corresponds to the enlargement of the present space-based measurement capabilities through the introduction of new missions to answer these emerging and urgent user requirements. After extensive consultation, six potential high-priority candidate missions (HPCMs) have been identified (ESA, 2019b): the Copernicus Hyperspectral Imaging Mission for the Environment (CHIME), the Copernicus Imaging Microwave Radiometer (CIMR), the Copernicus Anthropogenic Carbon Dioxide Monitoring (CO2M) mission, the Copernicus Polar Ice and Snow Topography Altimeter (CRISTAL), the Copernicus Land Surface Temperature Monitoring (LSTM) mission, and the L-band Synthetic Aperture Radar (ROSE-L).
The strategic, environmental, and socio-economic importance of the Arctic region has been emphasized by the European Union in their integrated policy for the Arctic (https://ec.europa.eu/environment/efe/news/integrated-eu-policy-arctic-2016-12-08_en, last access: 10 July 2020), including the Arctic Ocean and its adjacent seas. Considering the sparse population and the lack of transport links, a capacity for continuous monitoring of the Arctic environment with satellites is considered essential. In light of this and of the importance of the polar regions more widely, guiding documents have been prepared in an EC-led user consultation process: the Polar Expert Group (PEG) User Requirements for a Copernicus Polar Mission Phase 1 report (Duchossois et al., 2018a), hereafter referred to as the PEG 1 report, and the Phase 2 report on users' requirements (Duchossois et al., 2018b), hereafter referred to as the PEG 2 report.
The required geophysical parameters for the polar regions are summarized and prioritized in the PEG 1 report, which addresses objectives as defined in the EU Arctic policy communication, namely climate change, environmental safeguarding, sustainable development, and support to indigenous populations and local communities. Floating ice parameters were listed as the top priority for a polar mission considering the availability of existing Copernicus products and services, their needs for improvement (e.g. in terms of spatial resolution and accuracy), and the current level of their technical and/or scientific maturity. The specific parameters include sea ice extent, concentration, thickness, type, drift, and velocity as well as thin ice distribution, iceberg detection, drift and volume change, and ice shelf (the floating extension of the ice sheets) thickness and extent. These parameters were given top priority by the European Commission due to their key position in operational services such as navigation and marine operations, meteorological and seasonal prediction, and climate model validation. The PEG 1 report also stresses the importance of a measuring capability for mountain glaciers and ice caps, seasonal snow, ice sheets, oceans, fresh water, and permafrost.
The Global Climate Observing System (GCOS, 2011) has stated that actions should be taken to ensure continuation of altimeter missions over sea ice. They suggested continuation of satellite synthetic-aperture radar (SAR) altimeter missions with enhanced techniques for monitoring sea ice thickness to achieve capabilities to produce time series of monthly 25 km sea ice thickness with 0.1 m accuracy for polar regions. It was mentioned that near-coincident data would help resolve uncertainties in sea ice thickness retrieval. Such measurements could be achieved, for example, through close coordination between radar and laser altimeter missions. In addition to sea ice thickness, other sea ice parameters retrievable from SAR altimetry, such as ice drift, shear and deformation, leads, and ice ridging, were pointed to as observable for future improvement.
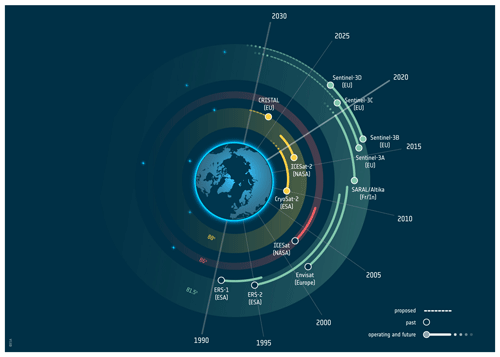
Figure 1Past, operating, approved, and proposed polar topography altimeter missions. By the mid-2020s, CRISTAL will fill the gap acquiring climate-critical data over polar ice north and south of 81.5∘ latitude (image: EOGB/ESA).
While the Copernicus Sentinel-3 mission provides partial altimetric measurements of the polar oceans, the satellites' inclination limits the coverage to latitudes between 81.5∘ N and 81.5∘ S. With the expected ongoing loss of Arctic sea ice, these satellites will monitor only a small amount of the Arctic ice cover during summer periods by the mid-2020s (see e.g. Quartly et al., 2019). Currently, the ESA's CryoSat-2 (Drinkwater et al., 2004; Wingham et al., 2006; Parrinello et al., 2018) is the only European satellite to provide monitoring of the oldest, thickest multi-year ice. However, continued monitoring of the polar regions – and the Arctic Ocean north of 81.5∘ N in particular – is at risk since CryoSat-2 has been operating in its extended mission scenario since its nominal end-of-mission lifetime of October 2013 (see Fig. 1). This risk has widely been recognized by the polar and ocean surface topography community. For example, at the 2019 Ocean Surface Topography Science Team (OSTST) meeting (Chicago, IL, USA, 21–25 October 2019) a recommendation was recorded (in view of the preparations for CRISTAL and other missions currently in operation) “to minimize likelihood of a gap in polar ocean and ice monitoring, the OSTST encourages Agencies to strive to launch a high-resolution polar altimeter in the early 2020s (such as the proposed HPCM CRISTAL) and to maintain operation of CryoSat-2, ICESat-2, and SARAL/AltiKa as long as possible”.
Based on the user requirements and priorities outlined in the PEG 1 report, a set of high-priority mission parameters were defined by the ESA's CRISTAL Mission Advisory Group (MAG) and the ESA, which led to the CRISTAL mission objectives (Table 1). The primary objectives drive the design and performance specifications of the CRISTAL mission, whereas the secondary objectives reflect the opportunity to support a wider range of users and services.
By addressing these objectives, the mission responds to a number of required parameters of interest and applications in Copernicus services. A mapping of the services to the parameters of interest and applications is listed in Table 2.
The following sections describe the key contributions of the mission in more detail, including the key requirements that guide the implementation of the mission.
4.1 Sea ice freeboard and thickness
Sea ice plays a critical role in Earth's climate system since it provides a barrier between the ocean and atmosphere, restricting the transfer of heat between the two. Due to its high albedo, the presence of sea ice reduces the amount of solar energy absorbed by the ocean. Arctic sea ice rejects brine during formation and fresh water during melting, and it is therefore a driving force of the global thermohaline circulation as well as the stratification of the upper layer of the ocean. The sea ice provides a critical habitat for marine mammals and for biological activity (e.g. Tynan et al., 2009), and it is a platform that enables subsistence hunting and travel for indigenous coastal communities.
The sea ice cover of the Arctic Ocean is waning rapidly. By 2019, the decline in September Arctic sea ice extent was about 13 % per decade relative to the 1981–2010 average, and the older, thicker, multi-year ice cover comprised ∼ 20 % of the winter ice pack compared to ∼ 45 % in the 1980s (Perovich et al., 2017; IPCC/SROCC, 2019). In the Southern Ocean sea ice is undergoing regional changes, with a decline observed in the Amundsen and Bellingshausen seas (Shepherd et al., 2018). These losses are having a profound impact on the climate, environment, and ecosystems of both polar regions. Monitoring the polar oceans is therefore of regional and global importance, and the long-term continuity of sea ice measurements is essential to extending both climate and operational data services.
Global warming and its Arctic amplification continue to contribute to the decrease in multi-year ice in the central Arctic Ocean (north of 81.5∘ N). It is therefore critical to obtain continuous, pan-Arctic observations of sea ice thickness, extending as close as possible to the North Pole. Continuous monitoring of Arctic Ocean sea ice conditions is necessary for safe navigation through ice-covered waters. When linked to previous measurements from Envisat, ICESat, CryoSat-2, and ICESat-2, the CRISTAL mission will deliver observations that provide a long-term record of sea ice thickness variability and trends that are critical to supporting climate services. Since sea ice thickness is an essential climate variable (see GCOS, 2011), its continuous measurement is required to understand the Arctic system and how ice loss is impacting global climate.
Shipping in ice-covered Arctic waters has increased significantly in recent years and is expected to continue to do so over the coming decades (IPCC/SROCC, 2019). In addition to traditional maritime operations and fishing in the high Arctic, several polar-class cruise liners are under construction. This means an increase in the need and scope of operational ice information services. A primary data source for national ice services is currently synthetic-aperture radar (SAR) imagery, specifically data acquired by Sentinel-1A and Sentinel-1B, RADARSAT-2, and the RADARSAT Constellation Mission. Thus, independent measurements of sea ice thickness distribution at reasonable latencies provided by CRISTAL will complement existing SAR measurements and benefit operational ice charting. Furthermore, observed sea ice thickness or freeboard distributions can be assimilated into sea ice models to generate ice forecasts needed for ice navigation and offshore operations.
Historically, satellite observations had primarily been used to monitor ice extent until Laxon et al. (2003) produced the first Arctic-wide sea ice thickness estimates from European Remote Sensing (ERS) satellite radar altimetry. Since then, various methods for converting the received signal to physical variables have been established (Giles et al., 2008a; Laxon et al., 2013; Kurtz et al., 2014; Ricker et al., 2014; Price et al., 2015; Tilling et al., 2018; Hendricks et al., 2018). The capability to obtain an estimate of sea ice freeboard and thickness and convert it to estimates of ice volume has enabled scientists to better understand the changing Arctic ice cover. Most recently, sea ice freeboard has been estimated from both Ka- and Ku-band measurements (Armitage and Ridout, 2015; Guerreiro et al., 2016; Lawrence et al., 2018).
Most sea ice thickness products are currently provided on a 25 km grid (see e.g. Sallila et al., 2019, for an overview of different products currently available), which corresponds to the GCOS user requirements (GCOS, 2011) but does not meet the specified accuracy requirements of 0.1 m. The residual, systematic uncertainty in sea ice thickness is estimated to be 0.56–0.61 m for ICESat (Connor et al., 2013), and it is 0.6 m for CryoSat-2 observations over first-year ice and 1.2 m for those over multi-year ice (Ricker et al., 2014). The uncertainty in ice thickness derived from CryoSat-2 observations is driven mainly by the unknown penetration of the radar pulse into the snow layer as a result of variable snow properties (Nandan et al., 2017, 2020) as well as the choice of retracker (Ricker et al., 2014). Reference is also made to Mallett et al. (2020), who find that assumptions concerning the time evolution of overlying snow density can lead to underestimates of sea ice thickness from radar altimetry.
While the focus of the Copernicus programme is on the Arctic, comprising all areas north of the southernmost tip of Greenland (∼ 60∘ N), the parameters specified for polar regions should equally be provided for its southern counterpart, the Antarctic, as well as all non-polar snow- and ice-covered surfaces.
The requirements for CRISTAL are currently stated to provide sea ice freeboard with an accuracy of 0.03 m along orbit segments of less than or equal to 25 km during winter months and to provide meaningful freeboard measurements during summer months. Winter months are months from October to April in the Northern Hemisphere and from May to October in the Southern Hemisphere. The system shall be capable of delivering sea ice thickness measurements with a vertical uncertainty of less than 0.15 m along orbit segments ≤ 25 km in winter months and of providing meaningful sea ice thickness estimates during summer months. The along-track resolution of sea ice thickness measurements shall be at least 80 m. The uncertainty requirement for sea ice thickness comes with a caveat as the thickness uncertainty depends on the uncertainty of auxiliary products. In the case of CRISTAL, snow thickness will be measured by the system, but snow and ice densities will still have to be estimated by other means. In light of the current 0.2 m sea ice thickness uncertainty from CryoSat-2 data assessed by Tilling et al. (2018) for a gridded, monthly product and the anticipated improvement from the dual-altimetry technology, especially in the snow depth and propagation estimates, a higher vertical uncertainty would seem reachable but requires further study. Currently, the retrieval accuracy of sea ice freeboard is limited by the range resolution of a radar altimeter. The large bandwidth of 500 MHz is an important driver for the CRISTAL instrument concept generation. A bandwidth of 500 MHz will improve the range resolution from 50 cm (as for CryoSat-2, with 320 MHz bandwidth) to ∼ 30 cm for CRISTAL. A radiometer will help in active–passive synergy to classify sea ice type (see e.g. Tran et al., 2009, for further justification).
4.2 Snow depth over sea ice
An accurate estimate of snow depth over Arctic sea ice is needed for signal propagation speed correction to convert radar freeboard to sea ice freeboard and freeboard to sea ice thickness (Laxon et al., 2003, 2013). The penetration aspects of a dual-frequency snow depth retrieval algorithm over Antarctica are complex (Giles et al., 2008b; Shepherd et al., 2018) and are not further elaborated here. In addition to uncertainty reduction for ice thickness and freeboard computation, the variation in snow depth is a parameter that is highly relevant for climate modelling, ice navigation, and polar ocean research. The snow climatology of Warren et al. (1999) is still the single most used estimate of snow depth in sea ice thickness processing (Sallila et al., 2019). The uncertainty in the original Warren et al. (1999) snow depth estimates is halved over first-year ice (Kurtz and Farrell, 2011; Zhou et al., 2020), but snow still represents the single most important contribution to uncertainty in the estimation of sea ice thickness and volume (Tilling et al., 2018). The studies of Lawrence et al. (2018) and Guerreiro et al. (2016) show the possibility of using Ku- and Ka-bands in mitigating the snow depth uncertainty. Dual-frequency methods improve the ability to reduce and estimate the uncertainties related to snow depth and sea ice thickness retrieval. The modelling community is particularly interested in the uncertainty information according to the user requirement study in the PEG 1 report. Having better abilities to estimate the related uncertainties improves prediction quality assessment of annual snowmelt over Arctic sea ice (Blockley and Peterson, 2018). The stratigraphy and electromagnetic properties of the snow layer contrast with those of the underlying ice and can be exploited to retrieve information on the snow layer properties if contemporaneous measurements are acquired from multiple scattering horizons (for details see Giles et al., 2007, who demonstrated the propagating uncertainties associated with snow depth and other geophysical parameters). A dual-frequency satellite altimeter, as proposed for the CRISTAL mission, will address this need. CRISTAL aims to provide an uncertainty in snow depth retrieval over sea ice of less than or equal to 0.05 m. The additional Ka-band measurements, with a 500 MHz bandwidth, support the discrimination between the ice and snow interfaces.
4.3 Ice sheets, glaciers, and ice caps
Earth's land ice responds rapidly to global climate change. For example, melting of glaciers, ice caps, and ice sheets over recent decades has altered regional and local hydrological systems and has impacted sea levels and patterns of global ocean circulation. The Antarctic and Greenland ice sheets are Earth's primary freshwater reservoirs and, due to their progressive imbalance, have made an accelerating contribution to global sea level rise during the satellite era (Shepherd et al., 2018, 2019). Glaciers outside of the ice sheets constituted nearly one-third of all sea level rise over the past 2 decades (Gardner et al., 2013; Wouters et al., 2019) Although ice dynamical models have improved, future losses from the polar ice sheets remain the largest uncertainty in sea level projections. Due to their continental scale, remote location, and hostile climatic environment, satellite measurements are the only practical solution for spatially and temporally complete monitoring of the polar ice sheets.
Estimates of ice sheet surface elevation change provide a wealth of geophysical information. They are used as the basis for computing the mass balance and sea level contribution of ice sheets of both Greenland and Antarctica (McMillan et al., 2014, 2016; Shepherd et al., 2012), for identifying emerging signals of mass imbalance (Flament and Rémy, 2012; Wingham et al., 2009), and for determining the loci of rapid ice loss (Hurkmans et al., 2014; Sørensen et al., 2015). Through combination with regional climate and firn models of surface processes, surface elevation change can be used to isolate ice dynamical changes at the scale of individual glacier catchments (McMillan et al., 2016).
A unique and continuous record of elevation measurements is provided by radar altimeters dating back to 1992. The maps are typically delivered in (1) high-resolution (5–10 km) rates of surface elevation change (for single or multiple missions, typically computed as a linear rate of change over a period of several years to decades) and (2) frequently (monthly, quarterly) sampled time series of the cumulative change, averaged across individual glacier basins. In addition to being used to quantify rates of mass balance and sea level rise, they also have a range of other applications, such as detection of subglacial lake drainage (Siegert et al., 2016) and investigations of the initiation and speed of inland propagation of dynamic imbalance (Konrad et al., 2017) that provide valuable information relating to the underlying physical processes that drive dynamical ice loss.
CRISTAL will extend the decades-long record of the generation of elevation measurements provided by radar altimeters. It will produce maps of ice surface elevation with an uncertainty of 2 m (the vertical accuracy threshold is 2 m, an absolute accuracy of 0.5 m can be assumed, and there is a relative accuracy goal of 0.2 m). The system shall be capable of delivering surface elevation with an along-track resolution of at least 100 m and a monthly temporal sampling. CRISTAL will be capable of tracking steep terrain with slopes less than 1.5∘ using its SARIn (interferometric synthetic-aperture radar) mode. High-resolution swath processing over ice sheets (about 5 km wide) can reveal complex surface elevation changes related to climate variability and ice dynamics as well as subglacial geothermal and magmatic processes (see e.g. Foresta et al., 2016). Elevation measurements of regions with smaller glaciers are often missing in CryoSat-2 data. Indeed, tracking algorithms are not efficient when rough terrain is encountered. Improvement in the tracking over glaciers is thus a key element in the instrument concept generation.
4.4 Sea level and coastal and inland water
Over the years and through constant improvement of the data quality, satellite altimetry has been used in a growing number of applications in Earth sciences. The altimeter measurements are helping us to understand and monitor the ocean: its topography, dynamics, and variability at different scales. Satellite observations for studying, understanding, and monitoring the ocean are more than essential over polar areas, where in situ data networks are very sparse and where profound and dramatic changes occur. This has also been expressed and emphasized by the Copernicus Marine Environmental Monitoring Service (CMEMS) as “ensuring continuity (with improvements) of the CryoSat-2 mission for sea level monitoring in polar regions” (CMEMS, 2017). “Reliable retrieval of sea level in the sea ice leads to reach the retrieval accuracy required to monitor climate change” is another CMEMS recommendation for polar and sea ice monitoring (see CMEMS, 2017).
Current data from the CMEMS catalogue do not allow a satisfactory sampling north of 81.5∘ N. It is of prime importance that the CRISTAL orbit configuration allows measurement coverage of the central Arctic Ocean with an omission not exceeding 2∘ of latitude around the poles. Sea level anomalies (SLAs) over frozen seas can only be provided by measurements in the leads. CRISTAL will contribute to the observation system for global observation of mean sea level, (sub-)mesoscale currents, wind speed, and significant wave height as a critical input to operational oceanography and marine forecasting services, and it will support sea ice thickness retrieval in the Arctic.
The high-inclination orbit of CRISTAL associated with high-resolution SAR and SARIn bi-band altimetry measurements would considerably extend our monitoring capability over the polar oceans. The development of tailored processing algorithms should not only have to track the low-frequency sea level trend in the presence of sea ice and to characterize large-scale and mesoscale ocean variations over regions not covered by conventional ocean altimeters. Beyond the observations of ice elevation variations, CRISTAL would offer the unique opportunity to improve our knowledge of the mutual ocean–cryosphere interactions over short- and long-term timescales for both poles. Southern Ocean circulation plays a key role in shaping the Antarctic cryosphere environment. First, it regulates sea ice production: as sea ice forms and ejects brine into the ocean, the ocean destabilizes and warms submerged waters that reach the ocean surface, limiting further ice production. Second, it impacts Antarctic ice sheet melt when warm and salty ocean currents access the base of floating glaciers through bathymetric troughs of the Antarctic continental shelf. These ocean currents melt the ice shelves from below and are the main causes of the current decline in floating ice shelves (Shepherd et al., 2019; Smith et al., 2020). Thus melting of ice shelves represents one of the largest uncertainties in the current prediction of global sea level change (Edwards et al., 2019), creating a major gap in our ability to respond and adapt to future climate change. Tightly linked with glacier melt, polar shelf circulation and its interaction with large-scale circulation also control the rate of bottom water production and deep-ocean ventilation, which impact the world's oceans on a timescale ranging from decades to millennia. Therefore, with a designed operational lifetime of at least 7.5 years (including in-orbit commissioning), the observation from the same sensor of each component of these multi-scale ice–ocean interactions would make CRISTAL unique in its capability to address climate issues of regional and global relevance. Over oceans, a secondary objective for the mission, the satellite will be able to measure sea surface height with an uncertainty of less than 3 cm. The main advantages and drawbacks of the Ka-band over the oceanic surface have been reviewed in Bonnefond et al. (2018). Given its high along-track resolution of less than 10 km and high temporal resolution of sea level anomalies, the mission can further contribute a suite of sea level products including sea surface height and mean sea surface (vertical accuracy in sea level anomaly retrieval of less than 2 cm is requested). The radiometer on board CRISTAL corrects the satellite altimeter data for the excess path delay resulting from tropospheric humidity. The microwave radiometer measurements will complement wet tropospheric corrections derived from numerical weather prediction and non-collocated atmospheric data from other satellite instruments to help meet the range accuracy requirement (Picard et al., 2015; Legeais et al., 2014; Vieira et al., 2019).
Observation of water level at the (Arctic) coast as well as of rivers and lakes is a key quantity in hydrological research (e.g. Jiang et al., 2017). Rivers and lakes not only supply fresh water for human use, including agriculture, but also maintain natural processes and ecosystems. The monitoring of global river discharge and its long-term trend contributes to the monitoring of global freshwater flux, which is critical for understanding the mechanism of global climate change. Satellite radar altimetry is a promising technology to do this on a regional to global scale. Satellite radar altimetry data have been used successfully to observe water levels in lakes and (large) rivers and have also been combined with hydrologic and hydrodynamic models. Combined with gravity-based missions like the NASA and Deutsches Zentrum für Luft- und Raumfahrt (DLR) GRACE and GRACE-FO missions, the joint use of the data will give information for ground water monitoring in the future.
4.5 Icebergs
Iceberg detection, volume change, and drift have been listed as a priority user requirement (Duchossois et al., 2018a, b).
Icebergs present a significant hazard to marine operations. Detection of icebergs in open water and in sea ice generally places a priority on wider satellite swaths to obtain greater geographic coverage. There is a need for automatic detection of icebergs for the safety of navigation and chart production. Iceberg concentration is given in CMEMS' catalogue at 10 km resolution covering Greenland waters. SAR imagery is the core input for iceberg detection. However, iceberg detection (in particular small icebergs) is also possible using high-resolution altimeter waveforms. Tournadre et al. (2018) demonstrated detection of icebergs from CryoSat-2 altimeter data using several modes and mention promising results with the Sentinel-3 data, which would be fed into a comprehensive dataset already built as part of the ALTIBERG project (Tournadre et al., 2016). The volume of an iceberg is valuable information for operational services and climate monitoring. For climate studies, the freshwater flux from the volume of ice transported by icebergs is a key parameter, with large uncertainties related to the volume of the icebergs. Measuring volume is currently only possible with altimetry by providing the iceberg freeboard elevation from the ocean surface. Iceberg volume has been calculated with altimetry with Envisat, Jason-1, and Jason-2 (e.g. Tournadre et al., 2015).
CryoSat-2 tracking over icebergs is operational, but icebergs with high freeboards may be missed in the current range window. The range window definition for CRISTAL is defined in order to ensure that echoes from icebergs are correctly acquired. In-flight performances for the measurement of the angle of arrival from CryoSat-2 are around 25 arcsec. An equivalent performance is necessary to retrieve across-track slopes and elevations. The CRISTAL design of the instrument and the calibration strategy will be designed to comply with the specification of 20 arcsec. CRISTAL will provide the unprecedented capability to detect icebergs at a horizontal resolution (gridded product) of at least 25 m. The products will be produced every 24 h in synergy with other high-resolution data such as SAR imagery. Iceberg distribution and volume products will be produced at 50 km resolution (gridded) on a monthly basis.
4.6 Snow on land and permafrost
CRISTAL may support and contribute to studies and services in relation to seasonal snow cover and permafrost applications over land. These are considered a secondary objective for the mission. The ability to retrieve snow depth with Ku- and Ka-band altimetry is limited over land (Rott et al., 2018). Snow studies over land area are so far largely limited to scatterometer when the Ku-band is used; examples of such retrievals are reviewed in Bartsch (2010). Measurements as provided by CRISTAL may, however, be useful in retrieving internal properties of the snowpack such as the existence of ice layers (e.g. due to rain on snow; Bartsch et al., 2010). The relevant properties of an upper snow layer contrast with those of an underlying ice layer (see also Sect. 4.2). Further, snow structure is reflected in differences observed in radar observations using different frequencies (Lemmetyinen et al., 2016). Snow structure anomalies as well as land surface state (freeze and thaw) are expected to be identified by time series analyses as such processes alter penetration depth. Altimeter data are also rarely used for permafrost studies. Such data can also be applied for monitoring lake level as a proxy for permafrost change (Zakharova et al., 2017). Surface status is closely interlinked with ground temperature (e.g. Kroisleitner et al., 2018), but usage of satellite altimetry in this context remains unexplored. Signal interaction with vegetation limits the applicability of Ku- and Ka-bands for soil observations regarding freeze and thaw status (Park et al., 2011) as well as surface height. Wider use of altimetry for snow and permafrost applications requires higher spatial resolution and temporal coverage than what is available to date. An improvement regarding the latter issues is expected with CRISTAL, which will expand the utility of altimeter observations for permafrost and snow monitoring over land.
This section summarizes the envisaged primary payload components to address the CRISTAL mission objectives. The design draws from the experience of several in-orbit missions in addition to the ongoing developments within the Sentinel-6 and MetOp-SG programmes and has a 7.5-year lifetime. CRISTAL's primary payload complement consist of the following:
-
A synthetic-aperture radar (SAR) altimeter operating at Ku-band and Ka-band centre frequencies is used for global elevation and topographic retrievals over land and marine ice, ocean, and terrestrial surfaces (see Figs. 2 and 3). In Ku-band (13.5 GHz), the SAR altimeter can also be operated in interferometric (SARIn) mode to determine across-track echo location. The Ka-band channel (35.75 GHz) has been introduced to improve snow depth retrievals over sea ice (see e.g. Guerreiro et al., 2016). A (vertical) range resolution of about 31 cm will be achieved to enhance freeboard measurement accuracy. Furthermore, a high along-track resolution of about 20 m is envisaged to improve ice floe discrimination. Heritage missions include CryoSat-2 (SAR/Interferometric Radar Altimeter, SIRAL), Sentinel-6 (Poseidon-4), and SARAL (Satellite with ARgos and ALtiKa). The CRISTAL altimeter (IRIS) is based on Poseidon-4 (Sentinel-6) and SIRAL (CryoSat-2) together with the addition of a Ka-band channel (analogous to AltiKa) and a bandwidth of 500 MHz (at both frequencies) to meet the improved range resolution requirement in comparison to heritage altimeters. It has the capability for fully focused SAR processing for enhanced along-track resolution by means of resolving full scatterer phase history (Egido and Smith, 2017). Digital processing will be implemented including matched filter range compression and on-board range cell migration (RCM) compensation by means of a range migration compensation (RMC) mode for on-board data reduction (heritage from Poseidon-4), reducing downlink load. With respect to the dual-frequency antenna (Ku- and Ka-band), an enhanced antenna mounting baseplate for improved baseline stability over CryoSat-2 will be required (20 arcsec vs. ∼ 30 arcsec for CryoSat-2).
-
A high-resolution passive microwave radiometer is included with the capability to provide data allowing retrievals of total column water vapour over the global ocean and up to 10 km from the coast (by means of improving the measurement system with high-frequency channels). The radiometer may also support cryosphere applications such as sea ice type classifications (Tran et al., 2009). Concerning the microwave instrument selection, potential options include a US Custom Furnished Item based on the National Aeronautics and Space Administration (NASA) Jet Propulsion Laboratory (JPL) AMR-C (Advanced Microwave Radiometer – Climate Quality), development of an EU high-resolution radiometer solution, and a two-channel solution derived from the Sentinel-3 microwave radiometer. The feasibility of each of these options will be further evaluated in the next mission phase (Phase B2 at the time of the system preliminary design review, expected late 2021).
-
A global navigation satellite system (GNSS) receiver compatible with both Galileo and Global Positioning System (GPS) constellations provides on-board timing, navigation, and provision of data for on-ground, precise orbit determination. Heritage GNSS solutions exist, such as those based upon the GPS- and Galileo-compatible Sentinel-1, Sentinel-2, Sentinel -3 C/D and Sentinel-6 A/B receivers. Precise Orbit Determination products will be provided by the Copernicus Precise Orbit Determination service.
-
A Laser Retroreflector Array (LRA) for use by the Satellite Laser Ranging network and by the International Laser Ranging Service for short-arc validation of the orbit. Heritage concepts suitable for CRISTAL include CryoSat-2 and Sentinel-3 LRAs.
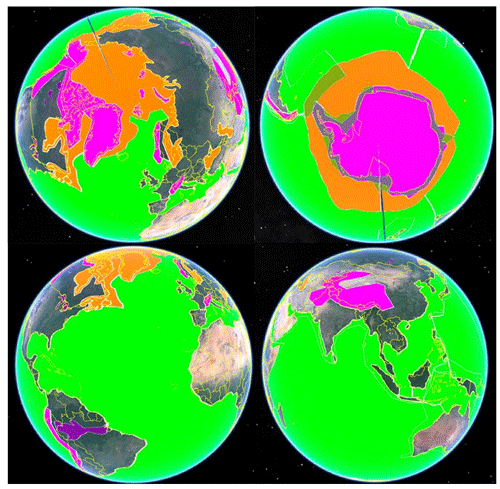
Figure 3Indicative mission geographic operating mode mask used in CRISTAL altimeter data volume sizing. Magenta: land ice, closed-burst SARIn mode, also including smaller ice caps; orange: sea ice and icebergs, open-burst SARIn mode (maximum coverage in Northern and Southern Hemisphere); green: open and coastal ocean, SARIn reduced window mode; purple: inland water (this is not anticipated as a mode but may be derived from one of the three key modes). Note: the wedge type feature in some of the images is an artefact of the display software.
Table 3Key altimeter characteristics in the different modes of operation (credits: Thales Alenia Space, France); n/a: not applicable.
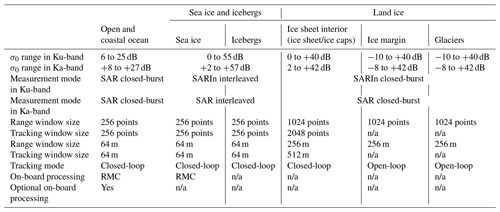
Three modes of radar operation are envisaged, which are automatically selected depending on the geographic location over the Earth's surface (see Table 3 and Fig. 3), prioritizing the retrieval of relevant geophysical parameters of interest.
-
Sea ice and iceberg mode: in Fig. 3, the proposed coverage is shown in orange. It is proposed that this mode makes a step forward in ice thickness retrieval by operating the instrument with the SAR interferometer configuration in Ku-band, i.e. a two-antenna cross-track interferometric principle. The measurement mode will be in an open-burst or interleaved arrangement in which receptions occur after each transmitted pulse. This results in an along-track resolution by ground processing to up to a few metres, which enables small sea ice sheets to be distinguished and narrow leads between them to be detected. The disadvantages of the open-burst transmission versus a closed-burst operation mode include a larger data volume and the power demand as well as variations in the pulse repetition frequency around the orbit. The interferometric operation allows the location of across-track sea ice leads, whilst open-burst timing allows full scatterer phase history reconstruction for fully focused processing (Egido and Smith, 2017). This improves sea ice lead discrimination (by means of improvement in sampling and resolution) and hence retrievals of elevation and polar SLAs by a significant factor (Armitage and Davidson, 2014). Open-burst Ka-band SAR is also provided to allow for improved retrieval of snow depth over sea ice.
-
Land ice mode: in Fig. 3, the proposed coverage is shown in magenta. Land ice elevation is retrieved by means of improved surface tracking based on the large range window. The accuracy of elevation retrievals is likely improved by a factor of 2 by means of increasing the number of echoes per unit time by a factor of 4 over the CryoSat-2 heritage design. The Ku-band SAR interferometer is used to retrieve the across-track point of closest approach supplemented with Ka-band SAR. Closed-burst operation (see e.g. Raney, 1998) is used over this surface type, in which the reflections arriving back at the radar are received after each transmitted burst has finished.
-
Open and coastal ocean mode: in Fig. 3, the proposed coverage shown in magenta provides Arctic and southern polar ocean retrieval of SLAs and precision SAR altimetry to complement other ocean topography missions including Sentinel-3, Sentinel-6, and next-generation topographic missions. In the case of open ocean, closed-burst SAR operation at Ku-band and Ka-band is used, and the RMC on-board processing is applied. This was first implemented in the frame of Sentinel-6, which provides a considerable gain in instrument data rate reduction. In addition, data will be collected over inland water regions using one of the above modes.
The latency of CRISTAL data products follows the requirements expressed in the PEG 1 and PEG 2 reports and provides measurements of different latencies according to the application need. The product latencies range from 3 h (some ocean Level 2 products) to 6 h (sea ice freeboard products), 24 h (sea ice thickness, sea ice snow depth, and iceberg detection products), 48 h (some ocean Level 1 and Level 2 products), and up to 30 d (surface elevation and some ocean Level 1 products). These data latencies indicate the time interval from data acquisition by the instrument to delivery as a Level 1B data product to the user.
CRISTAL directly addresses the EU Arctic policy and primary user requirements collected by the European Commission and provides sustained, long-term monitoring of sea ice thickness and land ice elevations. It thereby responds to needs for continuous pan-Arctic altimetric monitoring including the region of the Arctic Ocean north of 81.5∘ N. Antarctica will be equally well covered. The mission serves several key Copernicus operational services, in particular the Climate Change Service and Marine Environmental Monitoring Service, and makes contributions to the Land Monitoring Service, Atmospheric Monitoring Service, and Emergency Management Service.
CRISTAL will cover the polar regions with a Ku-band interferometric synthetic-aperture radar altimeter with supporting Ka-band channel. In addition, the payload contains a high- and low-frequency passive microwave radiometer to perform wet troposphere delay correction and surface-type classification over sea ice and ice sheets. The mission is designed for a 7.5-year design lifetime and will fly in an optimized orbit covering polar regions (omission ; weekly and monthly sub-cycles). A key element is the high along-track resolution (by ground processing up to a few metres when the novel interleaved SAR operation mode is used) to distinguish open ocean from sea ice surfaces. Thanks to the dual-frequency SAR altimetry capability, a snow depth product will be produced over sea ice with high accuracy in response to long-standing user needs.
CRISTAL has undergone and completed parallel preparatory (Phase A and B1) system studies in which mission and system requirements have been investigated and consolidated. The intermediate system requirement review has been completed with parallel industrial consortia compliant with the mission and system requirements. Next steps include the full definition, implementation, and in-orbit commissioning of CRISTAL (Phases B2, C/D, and E1), where a prototype and recurrent satellite will be developed.
AltiKa | Altimeter Ka-band |
AMR-C | Advanced Microwave Radiometer – Climate Quality |
C3S | Copernicus Climate Change Service |
Cal/Val | Calibration and validation |
CAMS | Copernicus Atmospheric Monitoring Service |
CEMS | Copernicus Emergency Management Service |
CGLS | Copernicus Global Land Service |
CIMR | Copernicus Polar Passive Microwave Imaging Mission |
CLS | Collecte Localisation Satellites |
CMEMS | Copernicus Marine Environmental Monitoring Service |
COP21 | United Nations Framework Convention on Climate Change, 21st Conference of the Parties |
CRISTAL | Copernicus Polar Ice and Snow Topography Altimeter |
CSC | Copernicus Space Component |
dB | Decibel |
EC | European Commission |
EO | Earth observation |
ESA | European Space Agency |
EU | European Union |
EUMETSAT | European Organisation for the Exploitation of Meteorological Satellites |
FMI | Finnish Meteorological Institute |
GCOS | Global Climate Observing System |
GMES | Global Monitoring for Environment and Security |
GNSS | Global navigation satellite system |
GPS | Global Positioning System |
IPCC | Intergovernmental Panel on Climate Change |
IRIS | Interferometric Radar Altimeter for Ice and Snow |
JPL | Jet Propulsion Laboratory |
LRA | Laser Retroreflector Array |
MetOp-SG | Meteorological Operational Satellite – Second Generation |
NASA | National Aeronautics and Space Administration |
OCO | Open and coastal ocean |
OSTST2019 | Ocean Surface Topography Science Team Meeting 2019 |
PEG | Polar Expert Group |
RADAR | Radio detection and ranging |
RCM | Range cell migration |
RMC | Range migration compensation |
SAR | Synthetic-aperture radar |
SARIn | Interferometric SAR |
SARAL | Satellite with ARgos and ALtiKa |
SIRAL | SAR/Interferometric Radar Altimeter |
SLA | Sea level anomaly |
STC | Short-time critical |
The work described in this paper is the result of consultations with Copernicus users and services as well as the CRISTAL Mission Advisory Group. No specific datasets have been used.
MK, as ESA mission scientist, is responsible for the mission requirements for the CRISTAL mission and was responsible for the overall conceptualization and structure of the paper. He drafted the manuscript and completed revisions based on co-author contributions and review. RC led the CRISTAL technical activities and contributed to the system concept description in Sect. 5. BB, JB, TC, MRD, AG, AL, ML, RM, INT, TP, and GR were involved in the supporting scientific and campaign activities or in the technical activities with industry. They contributed to Sects. 5 and 6 of this paper and to the overall prepublication critical review of the work. EA and CMP described and provided input and critical review of Sects. 1 and 2, which pertain mostly to the European Commission and EUMETSAT's involvement in the mission preparation and set-up. OA, AB, SaF, SiF, SG, AG, AH, ER, AS, MRvdB, and JY were members of the ESA's Mission Advisory Group in Phase A and B1 and provided input, critical review, and assistance with the manuscript.
The authors declare that they have no conflict of interest.
The authors would like to acknowledge the industrial and scientific teams involved in the Phase A and B1 study of the CRISTAL mission, significantly contributing to the success of the mission preparation in this feasibility phase. The authors would like to thank the anonymous reviewers, Alex Gardner, and the editor for their comments.
This paper was edited by Chris Derksen and reviewed by Alex Gardner and two anonymous referees.
Armitage, T. W. K. and Davidson, M. W. J.: Using the Interferometric Capabilities of the ESA CryoSat-2 Mission to Improve the Accuracy of Sea Ice Freeboard Retrievals, IEEE T. Geosci. Remote, 52, 529–536, https://doi.org/10.1109/TGRS.2013.2242082, 2014.
Bartsch, A.: Ten Years of SeaWinds on QuikSCAT for Snow Applications, Remote Sens., 2, 1142–1156, 2010.
Bartsch, A., Kumpula, T., Forbes, B. C., and Stammler, F.: Detection of snow surface thawing and refreezing in the Eurasian Arctic with QuikSCAT: implications for reindeer herding, Ecol. Appl., 20, 2346–2358, https://doi.org/10.1890/09-1927.1, 2010.
Blockley, E. W. and Peterson, K. A.: Improving Met Office seasonal predictions of Arctic sea ice using assimilation of CryoSat-2 thickness, The Cryosphere, 12, 3419–3438, https://doi.org/10.5194/tc-12-3419-2018, 2018.
Bonnefond, P., Verron, J., Aublanc, J., Babu, K.N., Bergé-Nguyen, M., Cancet, M., Chaudhary, A., Crétaux, J.-F., Frappart, F., Haines, B. J., Laurain, O., Ollivier, A., Poisson, J.-C., Prandi, P., Sharma, R., Thibaut, P., and Watson, C.: The Benefits of the Ka-Band as Evidenced from the SARAL/AltiKa Altimetric Mission: Quality Assessment and Unique Characteristics of AltiKa Data, Remote Sens., 10, 83, https://doi.org/10.3390/rs10010083, 2018.
Budyko, M. I.: The effect of solar radiation variations on the climate of the Earth, Tellus, 21, 611–619, https://doi.org/10.3402/tellusa.v21i5.10109, 1969.
Chen, J. L., Wilson, C. R., and Tapley, B. D.: Contribution of ice sheet and mountain glacier melt to recent sea level rise, Nat. Geosci., 6, 549–552, https://doi.org/10.1038/ngeo1829, 2013.
CMEMS-1: Copernicus Marine Environmental Monitoring System (CMEMS) requirements for the Evolution of the Copernicus Satellite Component, available at: http://marine.copernicus.eu/wp-content/uploads/2019/01/CMEMS-requirements-satellites.pdf (last access: 10 July 2020), 2017.
Connor, L. N., Farrell, S. L., McAdoo, D. C., Krabill, W. B., and Manizade S.: Validating ICESat Over Thick Sea Ice in the Northern Canada Basin, IEEE T. Geosci. Remote, 51, 2188–2200, https://doi.org/10.1109/TGRS.2012.2211603, 2013.
Copernicus Marine Environmental Monitoring Service (CMEMS): http://marine.copernicus.eu, last access: 10 July 2020.
Copernicus Land Monitoring Service (CLMS): http://land.copernicus.eu], last access: 10 July 2020.
Copernicus Atmospheric Monitoring Service (CAMS): https://atmosphere.copernicus.eu, last access: 10 July 2020.
Copernicus Emergency Management Service (CEMS): http://emergency.copernicus.eu, last access: 10 July 2020.
Copernicus Climate Change Service (C3S): http://climate.copernicus.eu, last access: 10 July 2020.
DeConto, R. M. and Pollard, D.: Contribution of Antarctica to past and future sea-level rise, Nature, 531, 591–597, 2016.
Drinkwater, M., Francis, R., Ratier, G., and Wingham, D.: The European Space Agency's Earth Explorer Mission CryoSat: Measuring variability in the cryosphere, Ann. Glaciol., 39, 313–320, https://doi.org/10.3189/172756404781814663, 2004.
Duchossois G., Strobl, P., Toumazou ,V., Antunes, S., Bartsch, A., Diehl, T., Dinessen, F., Eriksson, P., Garric, G., Houssais, M-N., Jindrova, M., Muñoz-Sabater J., Nagler T., and Nordbeck, O.: User Requirements for a Copernicus Polar Mission – Phase 1 Report, EUR 29144 EN, Publications Office of the European Union, Luxembourg, https://doi.org/10.2760/22832, 2018a.
Duchossois, G., Strobl P., Toumazou V., Antunes, S., Bartsch, A., Diehl, T., Dinessen, F., Eriksson, P., Garric, G., Holmlund, K., Houssais, M.-N., Jindrova, M., Kern, M., Muñoz-Sabater, J., Nagler, T., Nordbeck, O., and de Witte, E.: User Requirements for a Copernicus Polar Mission – Phase 2 Report EUR 29144 EN, Publications Office of the European Union, Luxembourg, https://doi.org/10.2760/44170, 2018b.
Edwards, T. L., Brandon, M. A., Durand, G., Edwards, N. R., Golledge, N. R., Holden, P. B., Nias, I. J., Payne, A. J., Ritz, C., and Wernecke, A.: Revisiting Antarctic ice loss due to marine ice-cliff instability, Nature, 566, 58–64, https://doi.org/10.1038/s41586-019-0901-4, 2019.
Egido, A. and Smith, W. H. F.: Fully Focused SAR Altimetry: Theory and Applications, IEEE T. Geosci. Remote, 55, 392–406, 2017.
ESA: Long-term Scenario, available at: https://www.copernicus.eu/sites/default/files/2019-01/Copernicus_Work_Programme_2019.pdf (last access: 10 July 2020), 2019a.
ESA: Copernicus High Priority Candidates, Mission Requirements Documents for the sixe candidate missions, available at: https://www.esa.int/Applications/Observing_the_Earth/Copernicus/Copernicus_High_Priority_Candidates (last access: 10 July 2020), 2019b.
European Commission: Copernicus evolution, Guidance Document for Horizon 2020 Work Programme 2018–2020, Copernicus evolution, LC-SPACE-02-EO-2018, LC-SPACE-03-EO-2018, available at: https://ec.europa.eu/research/participants/data/ref/h2020/other/guides_for_applicants/h2020-supp-info-space-2-3-18-20_en.pdf (last access: 10 July 2020), 2017.
Flament, T. and Rémy, F.: Dynamic thinning of Antarctic glaciers from along-track repeat radar altimetry, J. Glaciol., 58, 830–840, https://doi.org/10.3189/2012JoG11J118, 2012.
Foresta L., Gourmelen, N., Palsson, F., Nienow, P., Bjoernsson, H., and Shepherd, A.: Surface elevation change and mass balance of Icelandic ice caps derived from swath mode CryoSat-2 altimetry, Geophys. Res. Lett., 43, 12–138, https://doi.org/10.1002/2016GL071485, 2016.
Francis, J. A., Vavrus, S. J., and Cohen, J.: Amplified Arctic warming and mid-latitude weather: new perspectives on emerging connections, WIRES Clim. Change, 8, e474, https://doi.org/10.1002/wcc.474, 2017.
Gardner, A. S., Moholdt, G., Cogley J. G., Wouters, B., Arendt, A. A., Wahr, J. A., Berthier, E., Hock, R., Pfeffer, W. T., Kaser, G., Ligtenberg, S. R. M., Bolch, M. J., Sharp, M. J., Hagen, J. O., van den Broeke, M. R., and Paul, F.: A Reconciled Estimate of Glacier Contributions to Sea Level Rise: 2003 to 2009, Science, 340, 852–857, https://doi.org/10.1126/science.1234532. 2013.
GCOS: Systematic observation requirements for satellite-based products for climate 2011 update: Supplemental details to the satellite-based component of the “Implementation plan for the global observing system for climate in support of the UNFCCC (2010 update), GCOS Rep. 154, available at: https://library.wmo.int/index.php?lvl=notice_display&id=449#.XwhdGefLj-g (last access: 10 July 2020), 2011.
Giles, K. A., Laxon, S. W., Wingham, D. J., Wallis, D. W., Krabill, W. B., Leuschen, C. J., McAdoo, D., Manizade, S. S., and Raney, R. K.: Combined airborne laser and radar altimeter measurements over the Fram Strait in May 2002, Remote Sens. Environ., 111, 182–194, 2007.
Giles, K. A., Laxon, S. W., and Ridout, A. L.: Circumpolar thinning of Arctic sea ice following the 2007 record ice extent minimum, Geophys. Res. Lett., 35, L22502, https://doi.org/10.1029/2008GL035710, 2008a.
Giles, K. A., Laxon, S. W., and Worby, A. P.: Antarctic sea ice elevation from satellite radar altimetry, Geophys. Res. Lett., 35, L03503, https://doi.org/10.1029/2007GL031572, 2008b.
Gourmelen, N., Escorihuela, M. J., Shepherd, A., Foresta, L., Muir, A., Garcia-Mondejar, A., Roca, M., Baker, S. G., and Drinkwater, M. R.: CryoSat-2 swath interferometric altimetry for mapping ice elevation and elevation change, Adv. Space Res., 62, 1226–1242, https://doi.org/10.1016/j.asr.2017.11.014, 2017.
Guerreiro, K., Fleury, S., Zakharova, E., Rémy, F., and Kouraev, A.: Potential for estimation of snow depth on Arctic sea ice from CryoSat-2 and SARAL/AltiKa missions, Remote Sens. Environ., 186, 339–349, https://doi.org/10.1016/j.rse.2016.07.013, 2016.
Hendricks, S., Ricker, R., and Helm, V.: User Guide – AWI CryoSat-2 Sea Ice Thickness Data Product (v1.2), AWI, hdl:10013/epic.48201.d001, 2016.
Hurkmans, R. T. W. L., Bamber, J. L., Davis, C. H., Joughin, I. R., Khvorostovsky, K. S., Smith, B. S., and Schoen, N.: Time-evolving mass loss of the Greenland Ice Sheet from satellite altimetry, The Cryosphere, 8, 1725–1740, https://doi.org/10.5194/tc-8-1725-2014, 2014.
IPCC: IPCC Summary for Policymakers, in: IPCC Special Report on the Ocean and Cryosphere in a Changing Climate, edited by: Pörtner, H.-O., Roberts, D. C., Masson-Delmotte, V., Zhai, P., Tignor, M., Poloczanska, E., Mintenbeck, K., Nicolai, M., Okem, A., Petzold, J., Rama, B., and Weyer, N., available at: https://www.ipcc.ch/site/assets/uploads/sites/3/2019/11/03_SROCC_SPM_FINAL.pdf (last access: 10 July 2020), 2019.
Jiang, L., Schneider, R., Andersen, O. B., and Bauer-Gottwein, P.: CryoSat-2 Altimetry Applications over Rivers and Lakes, Water, 9, 211, https://doi.org/10.3390/w9030211, 2017.
Konrad, H., Gilbert, L., Cornford, S. L., Payne, A., Hogg, A., Muir, A., and Shepherd, A: Uneven onset and pace of ice-dynamical imbalance in the Amundsen Sea Embayment, West Antarctica, Geophys. Res. Lett., 44, 910–918, https://doi.org/10.1002/2016GL070733, 2017.
Kroisleitner, C., Bartsch, A., and Bergstedt, H.: Circumpolar patterns of potential mean annual ground temperature based on surface state obtained from microwave satellite data, The Cryosphere, 12, 2349–2370, https://doi.org/10.5194/tc-12-2349-2018, 2018.
Kurtz, N. T., Galin, N., and Studinger, M.: An improved CryoSat-2 sea ice freeboard retrieval algorithm through the use of waveform fitting, The Cryosphere, 8, 1217–1237, https://doi.org/10.5194/tc-8-1217-2014, 2014.
Lawrence, I. R., Tsamados, M. C., Stroeve, J. C., Armitage, T. W. K., and Ridout, A. L.: Estimating snow depth over Arctic sea ice from calibrated dual-frequency radar freeboards, The Cryosphere, 12, 3551–3564, https://doi.org/10.5194/tc-12-3551-2018, 2018.
Laxon, S. W., Peacock, N., and Smith, D.: High interannual variability of sea ice thickness in the Arctic region, Nature, 425, 947–950, 2003.
Laxon S. W., Giles, K. A., Ridout, A. L., Wingham, D. J., Willatt, R., Cullen, R., Kwok, R., Schweiger, A., Zhang, J., Haas, C., Hendricks, S., Krishfield, R., Kurtz, N., Farrell, S., and Davidson, M.: CryoSat-2 estimates of Arctic sea ice thickness and volume, Geophys. Res. Lett., 40, 732–737, https://doi.org/10.1002/grl.50193, 2013.
Legeais, J.-F., Ablain, M., and Thao, S.: Evaluation of wet troposphere path delays from atmospheric reanalyses and radiometers and their impact on the altimeter sea level, Ocean Sci., 10, 893–905, https://doi.org/10.5194/os-10-893-2014, 2014.
Lemmetyinen, J., Kontu, A., Pulliainen, J., Vehviläinen, J., Rautiainen, K., Wiesmann, A., Mätzler, C., Werner, C., Rott, H., Nagler, T., Schneebeli, M., Proksch, M., Schüttemeyer, D., Kern, M., and Davidson, M. W. J.: Nordic Snow Radar Experiment, Geosci. Instrum. Method. Data Syst., 5, 403–415, https://doi.org/10.5194/gi-5-403-2016, 2016.
Mallett, R. D. C., Lawrence, I. R., Stroeve, J. C., Landy, J. C., and Tsamados, M.: Brief communication: Conventional assumptions involving the speed of radar waves in snow introduce systematic underestimates to sea ice thickness and seasonal growth rate estimates, The Cryosphere, 14, 251–260, https://doi.org/10.5194/tc-14-251-2020, 2020.
McMillan, M., Shepherd, A., Sundal, A., Briggs, K., Muir, A., Ridout, A., Hogg, A., and Wingham, D.: Increased ice losses from Antarctica detected by CryoSat-2, Geophys. Res. Lett., 41, 3899–3905, https://doi.org/10.1002/2014GL060111, 2014.
McMillan, M., Leeson, A., Shepherd, A., Briggs, K., Armitage, T. W. K. T. W. K., Hogg, A., Munneke, P. K., van den Broeke, M., Noel, B., van den Berg, W. J., Ligtenberg, S., Horwarth, M., Groh, A. Muir, A., and Gilbert, L.: A high-resolution record of Greenland mass balance, Geophys. Res. Lett., 43, 7002–7010, https://doi.org/10.1002/2016GL069666, 2016.
Meredith, M., Sommerkorn, M., Cassotta, S., Derksen, C., Ekaykin, A., Hollowed, A., Kofinas, G., Mackintosh, A., Melbourne-Thomas, J., Muelbert, M. M. C., Ottersen, G., Pritchard, H., and Schuur, E. A. G.: Polar regions, in: IPCC Special Report on the Ocean and Cryosphere in a Changing Climate, edited by: Pörtner, H.-O., Roberts, D. C., Masson-Delmotte, V., Zhai, P., Tignor, M., Poloczanska, E., Mintenbeck, K., Alegría, A., Nicolai, M., Okem, A., Petzold, J., Rama, B., and Weyer, N. M., IPCC, 2019.
Nandan, V., Geldsetzer, T., Yackel, J., Mahmud, M., Scharien, R., Howell, S., King, J., Ricker, R., and Else, B.: Effect of snow salinity on CryoSat-2 Arctic first-year sea ice freeboard measurements, Geophys. Res. Lett., 44, 10419–10426, https://doi.org/10.1002/2017GL074506, 2017.
Nandan, V., Scharien, R. K., Geldsetzer, T., Kwok, R., Yackel, J. J., Mahmud, M. S., Roesel, A., Tonboe, R., Granskog, M., Willatt, R., Stroeve, J., Nomura, D., and Frey, M.: Snow Property Controls on Modelled Ku-band Altimeter Estimates of First-Year Sea Ice Thickness: Case studies from the Canadian and Norwegian Arctic, IEEE J. Sel. Top Appl., 13, 1082–1096, https://doi.org/10.1109/jstars.2020.2966432, 2020.
Park, S.-E., Bartsch, A., Sabel, D., Wagner, W., Naeimi, V., and Yamaguchi, Y. : Monitoring freeze/thaw cycles using ENVISAT ASAR global mode, Remote Sens. Environ., 115 , 3457–3467, https://doi.org/10.1016/j.rse.2011.08.009, 2011.
Parrinello, T., Shepherd, A., Bouffard, J., Badessi, S., Casal, T., Davidson, M., Fornari, M., Maestroni, E., and Scagliola, M.: CryoSat: ESA's ice mission – Eight years in space, Adv. Space Res., 62, 1178–1190, https://doi.org/10.1016/j.asr.2018.04.014, 2018.
Perovich, D., Meier, W., Tschudi, M., Farrell, S., Gerland, S., Hendricks, S., Krumpen, T., and Haas, C.: Sea ice cover: in “State of the Climate in 2016”, B. Am. Meteorol. Soc., 98, S131–S133, 2017.
Picard, B., Frery, M. L., Obligis, E., Eymard, L., Steunou, N., and Picot, N.: SARAL/AltiKa Wet Tropospheric Correction: In-Flight Calibration, Retrieval Strategies and Performances, Marine Geodesy, 38, 277–296, https://doi.org/10.1080/01490419.2015.1040903, 2015.
Price, D., Beckers, J., Ricker, R., Kurtz, N., Rack, W., Haas, C., Helm, V., Hendricks, S. Leonard, G., and Langhorne, P. J.: Evaluation of CryoSat-2 derived sea-ice freeboard over fast ice in McMurdo Sound, Antarctica, J. Glaciol., 61, 285–300, https://doi.org/10.3189/2015JoG14J157, 2015.
Prowse, T., Alfredsen, K., Beltaos, S., Bonsal, B., Duguay, C., Korhola, A., McNamara, J., Pienitz, R., Vincent, W. F., Vuglinsky, V., and Weyhenmeyer, G. A: Past and Future Changes in Arctic Lake and River Ice, Ambio, 40, 53–62, https://doi.org/10.1007/s13280-011-0216-7, 2011.
Quartly, G. D., Rinne, E., Passaro, M., Andersen, O. A., Dinardo, S., Fleury S., Guillot, A., Hendricks, S., Kurekin, A. A., Müller, F. L., Ricker, R. , Skourup, H., and Tsamados, M.: Retrieving Sea Level and Freeboard in the Arctic: A Review of Current Radar Altimetry Methodologies and Future Perspectives, Remote Sens., 11, 881, https://doi.org/10.3390/rs11070881, 2019.
Raney, R. K.: The Delay/Doppler Radar Altimeter, IEEE T. Geosci. Remote, 36, 1578–1588, 1998.
Ricker, R., Hendricks, S., Helm, V., Skourup, H., and Davidson, M.: Sensitivity of CryoSat-2 Arctic sea-ice freeboard and thickness on radar-waveform interpretation, The Cryosphere, 8, 1607–1622, https://doi.org/10.5194/tc-8-1607-2014, 2014.
Rott, H., Shi, J., Xiong, C., and Cui, Y.: Snow properties from active remote sensing instruments, in: Comprehensive Remote Sensing, edited by: Liang, S., vol. 4, 237–257, https://doi.org/10.1016/B978-0-12-409548-9.10359-8, 2018.
Sallila, H., Farrell, S. L., McCurry, J., and Rinne, E.: Assessment of contemporary satellite sea ice thickness products for Arctic sea ice, The Cryosphere, 13, 1187–1213, https://doi.org/10.5194/tc-13-1187-2019, 2019.
Screen, J. A. and Simmonds, I.: The central role of diminishing sea ice in recent Arctic temperature amplification, Nature, 464, 1334–1337, https://doi.org/10.1038/nature09051, 2010.
Serreze, M. C. and Francis, J. A.: The Arctic Amplification Debate, Clim. Change, 76, 241–264, https://doi.org/10.1007/s10584-005-9017-y, 2006.
Siegert, M. J., Ross, N., and Le Brocq, A. M.: Recent advances in understanding Antarctic subglacial lakes and hydrology, Philos. T. R. Soc. A, 374, 2059, https://doi.org/10.1098/rsta.2014.0306, 2016.
Shepherd, A., Ivins, E. R., Geruo, A., Barletta, V. R., Bentley, M. J., Bettadpur, S., Briggs, K. H., Bromwich, D. H., Forsberg, R., Galin, N., Horwath, M., Jacobs, S., Joughin, I., King, M. A., Lenaerts, J. T. M., Li, J., Ligtenberg, S. R. M., Luckman, A., Luthcke, S. B., McMillan, M., Meister, R., Milne, G., Mouginot, J., Muir, A., Nicolas, J. P., Paden, J., Payne, A. J., Pritchard, H., Rignot, E., Rott, H., Sandberg Sørensen, L., Scambos, T.A., Scheuchl, B., Schrama, E. J. O., Smith B., Sundal, A. V., van Angelen, J. H., van de Berg, W. J., van den Broeke, M. R., Vaughan, D. G., Velicogna, I., Wahr, J., Whitehouse, P. L., Wingham, D. J., Yi, D., Young, D., and Zwally, H. J.: A reconciled estimate of ice-sheet mass balance, Science, 338, 6111, https://doi.org/10.1126/science.1228102, 2012.
Shepherd, A., Fricker, H. A., and Farrell, S. L.: Trends and connections across the Antarctic cryosphere, Nature, 558, 223–232, https://doi.org/10.1038/s41586-018-0171-6, 2018.
Shepherd, A., Ivins, E., Rignot, E., et al.: Mass balance of the Greenland Ice Sheet from 1992 to 2018, Nature, 579, 233–239, https://doi.org/10.1038/s41586-019-1855-2, 2020.
Shiklomanov, A. I., Lammers, R. B., Rawlins, M. A., Smith, L. C., and Pavelsky, T. M.: Temporal and spatial variations in maximum river discharge from a new Russian data set, J. Geophys. Res.-Biogeo., 112, G04S53, https://doi.org/10.1029/2006JG000352, 2007.
Sørensen, L. S., Simonsen, S. B., Meister, R., Forsberg, R., Levinsen, J. F., and Flament, T.: Envisat-derived elevation changes of the Greenland ice sheet, and a comparison with ICESat results in the accumulation area, Remote Sens. Environ., 160, 56–62, https://doi.org/10.1016/j.rse.2014.12.022, 2015.
Smith, B., Fricker, H. A., Gardner, A. S., Medley, B., Nilsson, J., Paolo, F. S., Holschuh, N., Adusumilli, S., Brunt, K., Csatho, B., Harbeck, K., Markus, T., Neumann, T., Siegfried, M. R., and Zwally, H. J.: Pervasive ice sheet mass loss reflects competing ocean and atmosphere processes, Science, 368, 1239–1242, https://doi.org/10.1126/science.aaz5845, 2020.
Tilling, R. L., Ridout, A., and Shepherd, A.: Estimating Arctic sea ice thickness and volume using CryoSat-2 radar altimeter data, Adv. Space Res., 62, 1203–1225, https://doi.org/10.1016/j.asr.2017.10.051, 2018.
Tournadre, J., Bouhier, N., Girard-Ardhuin, F., and Rémy, F.: Large icebergs characteristics from altimeter waveforms analysis, J. Geophys. Res.-Oceans, 120, 1954–1974, https://doi.org/10.1002/2014JC010502, 2015.
Tournadre, J., Bouhier, N., Girard-Ardhuin, F., and Remy, F.: Antarctic icebergs distributions 1992–2014, J. Geophys. Res.-Oceans, 121, 327–349, 2016.
Tournadre, J., Bouhier, N., Boy, F., and Dinardo, S.: Detection of iceberg using Delay Doppler and interferometric Cryosat-2 altimeter data., Remote Sens. Environ., 212, 134–147, https://doi.org/10.1016/j.rse.2018.04.037, 2018.
Tran, N., Girard-Ardhuin, F., Ezraty, R., Feng, H., and Femenias, P.: Defining a Sea Ice Flag for Envisat Altimetry Mission, IEEE Geosci. Remote S., 6, 77–81, 2009.
Tynan, C. T., Ainley, D. G., and Stirling, I.: Sea ice: a critical habitat for polar marine mammals and birds, chap. 11, in: Sea Ice, 2nd edn., edited by: Thomas. D. N. and Dieckmann, G. S., Wiley, https://doi.org/10.1002/9781444317145.ch11, 2009.
United Nations Framework Convention on Climate Change: 21st Conference of the Parties,Conference of Parties, Paris, France, 7–8 December 2015, available at: http://www.cop21paris.org/ (last access: 10 July 2020), 2014.
Vieira, T., Fernandes, M. J., and Lázaro, S.: Impact of the New ERA5 Reanalysis in the Computation of Radar Altimeter Wet Path Delays, IEEE T. Geosci. Remote, 57, 9849–9857, https://doi.org/10.1109/TGRS.2019.2929737, 2019.
Warren, S. G., Rigor, I. G., Untersteiner, N., Radionov, V. F., Bryazgin, N. N., Aleksandrov, Y. I., and Colony, R.: Snow depth on Arctic sea ice, J. Climate, 12, 1814–1829, 1999.
Whiteman, G., Hope C., and Wadhams, P.: Climate science: Vast costs of Arctic change, Nature, 499, 401–403, 2013.
Wingham, D., Wallis, D., and Shepherd, A.: Spatial and temporal evolution of Pine Island Glacier thinning, 1995–2006, Geophys. Res. Lett., 36, L17501, https://doi.org/10.1029/2009gl039126, 2009.
Wingham, D. J., Francis, C. R., Baker, S., Bouzinac, C., Brockley, D., Cullen, R., de Chateau-Thierry, P., Laxon, S. W., Mallow, U., Mavrocordatos, C., Phalippou, L., Ratier, G., Rey, L., Rostan, F., Viau, P., and Wallis, D. W.: CryoSat: A mission to determine the fluctuations in Earth's land and marine ice fields, Adv. Space Res., 37, 841–871, https://doi.org/10.1016/j.asr.2005.07.027, 2006
Wouters, B., Gardner, A. S., and Moholdt, G.: Global glacier mass loss during the GRACE satellite mission (2002–2016), Front. Earth Sci., 7, 96, https://doi.org/10.3389/feart.2019.00096, 2019.
Zakharova, E. A., Kouraev, A. V., Stephane, G., Franck, G., Desyatkin, R. V., and Desyatkin, A. R.: Recent dynamics of hydro-ecosystems in thermokarst depressions in Central Siberia from satellite and in situ observations: Importance for agriculture and human life, Sci. Total Environ., 615, 1290–1304, https://doi.org/10.1016/j.scitotenv.2017.09.059, 2018.
Zakharova, E. A., Nielsen, K., Kamenev, G., and Kouraev, A.: River discharge estimation from radar altimetry: Assessment of satellite performance, river scales and methods, J. Hydrol., 583, 124561, https://doi.org/10.1016/j.jhydrol.2020.124561, 2020.
Zhou, L., Stroeve, J., Xu, S., Petty, A., Tilling, R., Winstrup, M., Rostosky, P., Lawrence, I. R., Liston, G. E., Ridout, A., Tsamados, M., and Nandan, V.: Inter-comparison of snow depth over sea ice from multiple methods, The Cryosphere Discuss., https://doi.org/10.5194/tc-2020-65, in review, 2020.
- Abstract
- Introduction
- Expansion and evolution of the Copernicus Space Component
- Objectives of the CRISTAL mission
- Key contributions of the CRISTAL mission
- CRISTAL mission concept
- Conclusions and CRISTAL mission status
- Appendix A: List of abbreviations
- Data availability
- Author contributions
- Competing interests
- Acknowledgements
- Review statement
- References
- Abstract
- Introduction
- Expansion and evolution of the Copernicus Space Component
- Objectives of the CRISTAL mission
- Key contributions of the CRISTAL mission
- CRISTAL mission concept
- Conclusions and CRISTAL mission status
- Appendix A: List of abbreviations
- Data availability
- Author contributions
- Competing interests
- Acknowledgements
- Review statement
- References