the Creative Commons Attribution 4.0 License.
the Creative Commons Attribution 4.0 License.
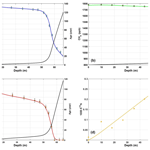
Very old firn air linked to strong density layering at Styx Glacier, coastal Victoria Land, East Antarctica
Youngjoon Jang
Sang Bum Hong
Christo Buizert
Hun-Gyu Lee
Sang-Young Han
Ji-Woong Yang
Yoshinori Iizuka
Akira Hori
Yeongcheol Han
Seong Joon Jun
Pieter Tans
Taejin Choi
Seong-Joong Kim
Soon Do Hur
Firn air provides plenty of old air from the near past, and can therefore be useful for understanding human impact on the recent history of the atmospheric composition. Most of the existing firn air records cover only the last several decades (typically 40 to 55 years) and are insufficient to understand the early part of anthropogenic impacts on the atmosphere. In contrast, a few firn air records from inland sites, where temperatures and snow accumulation rates are very low, go back in time about a century. In this study, we report an unusually old firn air effective CO2 age of 93 years from Styx Glacier, near the Ross Sea coast in Antarctica. This is the first report of such an old firn air age (>55 years) from a warm coastal site. The lock-in zone thickness of 12.4 m is larger than at other sites where snow accumulation rates and air temperature are similar. High-resolution X-ray density measurements demonstrate a high variability of the vertical snow density at Styx Glacier. The CH4 mole fraction and total air content of the closed pores also indicate large variations in centimeter-scale depth intervals, indicative of layering. We hypothesize that the large density variations in the firn increase the thickness of the lock-in zone and, consequently, increase the firn air ages because the age of firn air increases more rapidly with depth in the lock-in zone than in the diffusive zone. Our study demonstrates that all else being equal, sites where weather conditions are favorable for the formation of large density variations at the lock-in zone preserve older air within their open porosity, making them ideal places for firn air sampling.
- Article
(3525 KB) - Full-text XML
-
Supplement
(432 KB) - BibTeX
- EndNote
Bubbles trapped in ice cores preserve ancient air and allow direct measurements of the atmospheric composition in the past (e.g., Petit et al., 1999). However, it is difficult to obtain air samples over the past several decades from ice cores since the more recent air has not yet been completely captured into bubbles closed off from the atmosphere. In contrast, we can obtain recent records from the interstitial air in the porous, unconsolidated snow layer (firn) on top of glaciers and ice sheets (Schwander, 1989; Schwander et al., 1993). In addition, we can take advantage of the very large amount of firn air because it allows us to accurately analyze isotopic ratios of greenhouse gases and many trace gases such as synthetic chlorofluorocarbons (CFCs), hydrochlorofluorocarbons (HCFCs), and SF6 (Buizert et al., 2012; Laube et al., 2012). However, reported firn air ages date back only several decades at the sites where snow accumulation rates are relatively high (Table 1). Old firn air (>55 years) was observed only at sites where surface temperatures and snow accumulation rates are low such as the South Pole (Battle et al., 1996) and inland Antarctic Megadunes (Severinghaus et al., 2010) (Table 1); however, even under such circumstances very old firn air is not guaranteed, as demonstrated by Dome C (Table 1).
In the firn layer, air moves through the open pores and is occluded into the adjacent ice at total porosity of ∼0.1 (Schaller et al., 2017). Firn air moves downward with the adjacent ice (advection), but is furthermore mixed by diffusion and affected by thermal and gravitational fractionation (Craig et al., 1988; Johnsen et al., 2000; Severinghaus et al., 2001; Goujon et al., 2003). In addition, gradual bubble trapping in the firn affects the movement of the air. As a result, at each depth there is a gas age distribution (Schwander et al., 1993; Trudinger et al., 1997), rather than a single gas age. Therefore, studying firn air is also important for interpreting the record of ancient air trapped in ice cores.
The firn column is generally divided into three zones: convective, diffusive, and lock-in, depending on the mechanisms of firn air movement (Sowers et al., 1992). The gravitational enrichment in 15N of N2 is traditionally used to define the boundaries between these zones. The convective zone is the upper part of the firn where the air can ventilate with the overlying atmosphere. With stronger wind pumping, there can be a deeper convective zone (Kawamura et al., 2013). This zone has the same δ15N of N2 value as that of the atmosphere. The diffusive zone is located under the convective zone, where molecular diffusion is the dominant mechanism of trace gas transport in interstitial air (Blunier and Schwander, 2000). The age of the firn air increases slowly with depth in the diffusive zone because of continued gas exchange with atmospheric air via diffusion. Heavier isotopes are enriched with depth due to the gravitational fractionation in the diffusive layer. Thus, δ15N of N2 gradually increases with depth in the diffusive zone. In the lock-in zone (LIZ) below the diffusive zone, gas diffusion is strongly impeded, although the bubbles are not entirely closed. The top of the lock-in zone is called lock-in depth (LID), where the gravitational fractionation ceases, so that the δ15N of N2 becomes constant. The bottom of the LIZ is defined as the full close-off depth (zCOD), where all air bubbles are closed off and firn becomes sealed ice. The zCOD can be estimated in two different ways. First, we can calculate the zCOD from firn densification models. Typically, the close-off occurs when the density of ice reaches about 830 kg m−3 (Blunier and Schwander, 2000), equivalent to a critical porosity of around 0.1 (Schaller et al., 2017). Also, if temperature is known, the average density at close-off can be estimated from empirical relations (Martinerie et al., 1992). Second, the deepest position where air can be sampled from the firn column is commonly considered (just above) the zCOD. In theory, the zCOD is the depth at which all pores are closed, but it can be ambiguous to specify the zCOD in the field because firn air can be sampled at a slightly deeper depth than that of the shallowest impermeable snow layer due to the existence of permeable layers at deeper depths – this effect is due to density layering (Mitchell et al., 2015).
The gas ages in the LIZ increase with depth faster than in the diffusive zone. In the LIZ, firn air moves downward at nearly the same rate as the surrounding ice, and therefore the age of the air increases with depth at nearly the same rate as the age of ice increases.
The age of the firn air is directly related to the movement of the firn air. Firn air models help calculate the firn air age using some parameters such as temperature and accumulation rate. However, several studies found that layering also affects the movement of firn air (e.g., Mitchell et al., 2015; Schaller et al., 2017). This implies that physical properties of the ice may affect the age of the firn air as well.
With regard to the lock-in and close-off processes, recent studies have focused on snow layers and microstructure of the firn (Hörhold et al., 2011; Gregory et al., 2014; Mitchell et al., 2015; Schaller et al., 2017). Density variability on scales of millimeters to tens of centimeters is observed at all polar sites. Hörhold et al. (2011) demonstrate that density variability is caused by physical snow properties in the firn column. Several studies have dealt with how snow density variations affect the transport of firn air (Hörhold et al., 2011; Mitchell et al., 2015). Mitchell et al. (2015) showed that the firn layering can affect the closure of pores and the thickness of LIZ, but the relation between snow density variations and range of firn air ages was not quantitatively examined.
In this study, we present firn air composition and δ15N−N2 from Styx Glacier, East Antarctica, to better understand the role of snow density variations in the age of firn air. We also present X-ray density data with millimeter resolution and compare them with δ18Oice and the closed-pore air composition in the LIZ.
We hypothesize that large snow density variations make the LIZ thicker and facilitate preservation of old firn air at the Styx Glacier. This study will help us better understand how the snow density layers of firn column affect movement and preservation of firn air, and provide guidance on selecting good sites for future firn air studies.
2.1 Firn air sampling and gas mole fractions analysis
The firn air and ice core were collected at the Styx Glacier, East Antarctica (73∘51.10′ S, 163∘41.22′ E, 1623 m a.s.l.), in December of 2014 (Fig. 1). This site is located 85 km north of the Korean Jang Bogo Station in the Southern Cross Mountains near the Ross Sea (Han et al., 2015). The snow accumulation rate is ∼10 cm ice per year, calculated from the Styx16b ice chronology based on methane correlation and tephra age tie-point and thinning functions (Yang et al., 2018). The mean annual surface temperature was measured as −31.7∘ by borehole temperature logging at 15 m depth, 2 years after the ice core drilling (Yang et al., 2018). Table 1 lists the characteristics of the Styx Glacier and other firn air sampling sites. A total of 13 samples from the surface to 64.8 m depth were collected. The firn air sampling device was constructed, following the design of the University of Bern, Switzerland (Schwander et al., 1993). Three vacuum pumps (two diaphragm pumps and one metal bellows pump), several pressure gauges, stainless steel lines, and vacuum valves were housed in an aluminum case to transfer to the polar site. The pump system plays four major roles: (1) purging modern air from the bottom of a borehole, (2) inflating the bladder to block the deep firn layers from the atmosphere, (3) removing the contaminated air and extracting the firn air, and (4) transporting firn air to a CO2 analyzer for measurements of gas mole fractions and store it in firn air containers. The bladder system is designed to be lowered into the borehole to seal the deep firn layer(s) being sampled from the atmosphere. The bladder consists of a 4 m long rubber tube and metal caps on the top and bottom of the rubber tube. The bladder's external diameter is 119.5 mm and the internal diameter is 114.5 mm. The material of the tube is butyl rubber (BIIR), which can endure low temperatures, providing no risk of sample contamination.
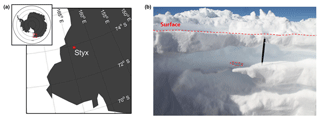
Figure 1(a) Location map of study site, Styx Glacier, Antarctica, and (b) a photo of surface snow density layers. The thickness of the snow density layers varies horizontally. The top boundaries of the high-density layers are sharp (horizontal red dashed line). A hole on a high-density layer surface is indicated by a red dashed circle. The length of the black sharp pencil in panel (b) is 14.3 cm.
The firn air samples were collected in 3 L glass flasks at all collection depths. However, to test preservation ability of the sample air containers, SilcoCan canisters were also used at four depths (0, 35.36, 43.42, 53.95 m). Accurate mole fractions of CO2, CH4, and SF6 were measured at the US National Oceanic and Atmospheric Administration (NOAA; https://www.esrl.noaa.gov/, last access: 30 August 2015). The results for the two types of containers show good agreement. δ15N of N2 was analyzed at Scripps Institution of Oceanography for correcting gravitational fractionation effect (Severinghaus et al., 2010).
2.2 Firn air transport model
We used the Center for Ice and Climate (CIC) firn air model which is a one-dimensional advection–diffusion model to simulate how the air moves in Styx firn column. In this model, there are four types of air transport in the open porosity: (1) molecular diffusion, (2) vigorous mixing in the convective zone, (3) advection, and (4) dispersion in the deep firn (Buizert, 2012; Buizert and Severinghaus, 2016). The model uses the stochastic bubble trapping formulation described by Mitchell et al. (2015).
2.3 CH4 in closed bubbles and total air content measurements
CH4 mole fraction in the (closed) air bubbles in the firn was measured at Seoul National University using a melt–refreeze air extraction method (Yang et al., 2017). A total of 124 discrete firn samples (cross section of 8.5 cm × 3 cm, length of 3 cm, ∼35 g) were prepared from four different depth intervals in the lock-in zone (54.59–55.34, 58.11–59.05, 59.86–60.55, 64.02–65.25 m). All ice samples were cut and trimmed by ∼2.5 mm with a band saw to remove contaminants on the surface ice. Then, the ice samples were inserted into the glass flasks attached to the gas extraction line. The pump system evacuated air in the flask placed in a cooled ethanol bath at −70∘ for 20 min. The evacuation time was limited to 20 min to prevent gas loss due to pore openings by sublimation. After the pressure dropped below 0.027 Pa, the ice samples in the glass flask were melted and air in the bubbles was extracted. After the melting was finished, we refroze the ice using a cooled ethanol bath to release the gas dissolved in the ice melt. Finally, the extracted air was injected into the sample loop of the gas chromatograph equipped with a flame ionization detector (FID). The calibration curve of the gas chromatography FID was calculated by the standard air prepared at NOAA with a CH4 mole fraction of 895 ppb on the NOAA04 scale (Dlugokencky et al., 2005).
Total air content of the firn ice samples was analyzed simultaneously with CH4 mole fraction using the wet extraction system at Seoul National University (SNU). The total air content was expressed as the volume of air trapped in the closed pores of unit mass of firn ice sample (in units of milliliters per gram of ice at STP conditions). The volume of air extracted from a firn ice sample was calculated by the ideal gas law with the internal pressure, volume, and temperatures of the sample flasks and vacuum lines. The pressure of extracted air was measured by a pressure manometer connected to the sample loop of the gas chromatography FID. As no direct measure of temperature was available, the temperature of extracted air was assumed to be identical to the surrounding temperatures; the ethanol temperature was used for the sample flasks, room temperature for vacuum lines, and valve box temperature (50 ∘C) for the sample loop. In this study, the corrections for bubble-cut effect and thermal gradient within vacuum line were not considered. A more detailed description of the protocols of total air content measurements is described in Yang (2019).
2.4 Analysis for stable isotopes of ice
After completing the measurements of the CH4 mole fraction in air, the meltwater was put into cleaned 125 mL bottles and analyzed for water stable isotope ratios at the Korea Polar Research Institute (KOPRI) using a cavity ring-down spectroscopy (CRDS, L1102-i, Picarro, USA) system. We performed the same analysis for the snow pit samples, but without CH4 analysis. The data are presented here as δ-notations:
The firn ice melt was filled into a 400 µL insert in a 2 mL glass vial using a syringe filter. The autosampler transported the ice melt samples in the insert to the vaporizer about 180 nL at a time. The samples with the liquid state were transferred to the cavity after being converted into the water vapor in a vaporizer at 110∘. The measurement precision evaluated by measuring an in-house standard repeatedly (n=12) was 0.08 ‰ (1σ standard deviation).
2.5 X-ray firn density measurement
We obtained high-resolution density data using the X-ray transmission method reported by Hori et al. (1999) for the firn ice at various depth intervals. This method is advantageous because it can measure continuously and nondestructively. The X-ray beam penetrates the ice samples, and the detector on the opposite side analyzes the intensity of the beam. To make equal thickness for each core section, upper and side parts of the half-circle-shaped core were shaved by a microtome. After putting the precut ice core on a rack, we set the rate of measurement at 50 mm min−1, and finally obtained 1 mm resolution density data.
3.1 Layered stratigraphy
We examined a snow pit, located 10 m away from the main ice core borehole, 2 years after drilling to understand the physical properties such as layers, density, and ice grain size of the upper firn at the Styx site. We scratched the snow wall by hand to remove soft layers and enhance the visibility of hard layers (Fig. 2a). The soft layers are presumed to be depth hoar, and the hard ones are wind crusts (Fig. 2b). The alternating layers repeat with intervals of a few centimeters to 20 cm. The top boundaries of the hard layers are sharp and extend horizontally about a meter, but the bottom boundaries are not well defined due to gradual density changes. 10 cm resolution density data were obtained by a density cutter (Proksch et al., 2016). The soft layers are coarsely grained, while the hard ones are finely grained (Fig. 2b–d).
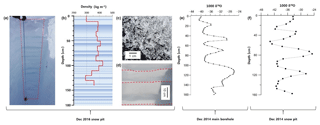
Figure 2Snow pit photos at Styx Glacier. (a) The snow pit with dimensions of 280 cm × 65 cm × 220 cm (length × width × height). (b) The illustration of qualitatively defined hard (dark blue) and soft (pale blue) layers observed in the top 180 cm depth interval. Progressive blue color changes indicate a gradual density decrease with depth. The red line is a 10 cm resolution density profile. (c) Coarse grains observed in a soft layer. The grains were placed on a black glove. (d) Enlarged snow layers. Dashed red lines indicate top boundaries of fine-grained hard layers. (e, f) Stable isotope ratio (δ18O) of snow profiles at the main core and a snow pit 100 m away from the main ice core borehole, respectively.
3.2 Firn gas sampling and the age of firn air
We calibrate the depth–diffusivity profile in the model using trace gases with a well-known atmospheric history (Buizert et al., 2012; Trudinger et al., 2013; Witrant et al., 2012). The atmospheric time series from well-dated firn air (MacFarling Meure et al., 2006) and instrument measurement records (NOAA; https://www.esrl.noaa.gov/) were used for calibration. The simulated mole fraction profiles match well with the observations (Fig. 3). CO2, CH4, SF6, and δ15N−N2 distributions in firn air were modeled. The model does not include thermal fractionation, and therefore provides a poor fit to the δ15N−N2 data in the upper firn where seasonal temperature gradients fractionate the gases. Fitting the barometric equation to the δ15N data of the upper diffusive zone suggests a convective zone thickness of approximately 3 m. This is within the typical range of observed convective zones, but perhaps lower than expected for a very windy site (Kawamura et al., 2006). The firn air age (black curves in Fig. 3) slowly increases with depth at the diffusive zone because it mixes with fresh atmospheric air on the surface mostly by molecular diffusion (Blunier and Schwander, 2000). In contrast, the firn air age rapidly increases within the LIZ at a rate similar to that of the ice age. The gas age distribution of Styx ice at zCOD is narrower than the other sites where old firn air is reported (Fig. 4); we simulate a spectral width of 15.9, 22.8, and 45.5 years at Styx, South Pole, and Megadunes, respectively. This means that the past atmospheric history of trace gases can in principle be reconstructed with higher resolution at Styx than at the other old-air firn sites.
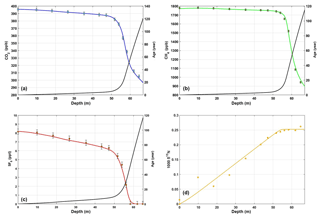
Figure 3CO2, CH4, and SF6 mole fractions and δ15N of N2 measurements (circles), as well as model results (solid line) for the Styx firn air (air in open porosity). Black lines are modeled ages for the gas species.
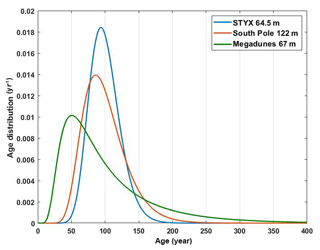
Figure 4Comparison of model-simulated CO2 age distributions at Styx (this study), South Pole (Battle et al., 1996), and Megadunes (Severinghaus et al., 2010).
We estimate the age of samples in two ways. First, after calibrating the firn air model, we can derive the mean sample age from the simulated gas age distribution. At the deepest Styx sampling depth (64.8 m) we simulate a mean CO2 age of 102 years and a mean CH4 age of 97 years; the CH4 age is younger than the CO2 age due to the higher diffusivity of CH4. Second, we can estimate the sample ages by comparing the measured trace gas concentrations directly to the atmospheric histories of these gases – this age has been called the “effective age” (Trudinger et al., 2013). The lowest CO2 mole fraction of 305.18 ppm at a depth of 64.8 m (304 ppm after correcting for gravitational enrichment) corresponds to the year 1921 and an effective age of 93 years (relative to sampling year 2014) on the Law Dome ice core record (MacFarling Meure et al., 2006; Rubino et al., 2019). The CH4 mole fraction of 943.36 ppb at the same depth (946.5 ppb after gravitational correction) corresponds to an effective age of 96 years (MacFarling Meure et al., 2006) (Fig. 3a, b). The second method provides younger ages because the growth rate in the atmospheric mixing ratios of these gases has increased over time, biasing the effective ages towards younger values (Trudinger et al., 2002). Table 1 lists effective CO2 ages in the deepest firn air sample for several sites; we here compare the effective CO2 age between sites rather than the modeled mean age, as it is purely empirical and does not rely on model assumptions.
Only a few firn air sites have effective CO2 ages around 93 years or older: 91 years from the South Pole (Battle et al., 1996) and 129 years from Megadunes (Severinghaus et al., 2010; Table 1). These sites are located in interior Antarctica and have low annual mean temperatures and low snow accumulation rates (Table 1). Firn densification takes a long time if snow accumulation and/or temperature are low, therefore firn air can be preserved for a long time without being trapped. In contrast, the Styx site is located near the coast and has relatively high snowfall, and therefore the age of 93 years is very unusual. Sites of comparable climate characteristics typically have an oldest firn air age of around 40 years. This indicates that there may be other factors that can permit preservation of the old firn air at Styx Glacier.
3.3 Density layering and its influence on bubble trapping
Firn density is the primary control on the bubble close-off process. Density layering leads to staggered bubble trapping, with high-density layers closing off before low-density ones (Stauffer et al., 1985; Etheridge et al., 1992; Mitchell et al., 2015; Rhodes et al., 2016).
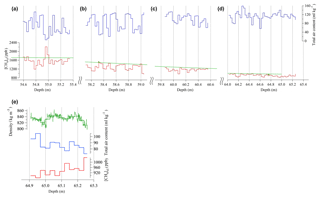
Figure 5(a–d) CH4 mole fraction in closed pores ([CH4]cl) (red line) and total air content (air volume per ice weight) (blue line) in the lock-in zone of Styx Glacier. (e) Comparison of density with [CH4]cl and total air content near zCOD. A small dashed-box in panel (d) indicates the depth interval of Fig. 5e.
Because the mole fractions of atmospheric greenhouse gases (CO2, CH4, N2O) have increased during the last century, we may obtain information on the timing of the closure of the bubbles from the greenhouse gas mole fractions of the air trapped in closed bubbles. In this study, we used the CH4 concentration in closed bubbles ([CH4]cl) and the total air content of the firn ice as indicators of the close-off process. The density and [CH4]cl show an anticorrelation (Fig. 5). Our results confirm the CH4 concentration-total air content relation observed in West Antarctic Ice Sheet (WAIS) Divide firn ice (Mitchell et al., 2015). High-density layers reach the lock-in and close-off densities at shallower depths than low-density layers. Thus, air bubbles are trapped at shallower depths in high-density layers. Early trapped bubbles preserve older air with lower greenhouse gas mole fractions. Higher air content is expected in the high-density layers, in which open porosity is small and closed porosity is large (Fig. 5). However, we cannot entirely exclude the possibility of some post-coring bubble close-off (Aydin et al., 2010). High open porosity in low-density layers may have more chances to trap modern ice storage air, which has a higher mole fraction of CH4 than atmospheric background levels.
Figure 5a shows [CH4]cl and total air content in the LIZ of the Styx firn. [CH4]cl generally decreases with depth and the centimeter-scale variability is reduced in the deep layers, while the total air content generally increases with depth. The [CH4]cl greater than CH4 mole fraction in neighboring firn air (green line in Fig. 5a–d) indicates part of bubbles formed after coring and increased the [CH4]cl, as previous studies also observed (Mitchell et al., 2015; Rhodes et al., 2013). Most [CH4]cl data show large centimeter-scale variations (Fig. 5). The highs and lows of [CH4]cl repeat with cycles of 6 to 24 cm (Fig. 5e). Note that the layering observed in the snow pit likewise shows irregular intervals (Fig. 2b). From the layer spacing, we conclude that bubble trapping at Styx is not controlled by annual layers (Sect. 4), as was observed at Law Dome (Etheridge et al., 1992).
The evolution of CH4 in the closed porosity may give information on how the snow layers can induce inhomogeneous records and help constrain the gas age distribution in ice (Fourteau et al., 2017). However, the details are beyond the scope of this study and we will focus on the firn air age in the open porosity.
3.4 High-resolution firn density measurements
The X-ray measurements show highly variable density on centimeter scales. We converted the high-resolution density to total porosity using the following equation:
where ρ is density of porous ice, ρice is density of bubble-free ice (919 kg m−3), and Φ is porosity. We test the idea that the lock-in zone corresponds to the depth range bounded by the first closed layer (porosity below 0.1) on the shallow side, and the last open layer (porosity above 0.1) on the deep side.
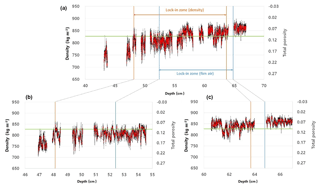
Figure 6High-resolution X-ray density data obtained from the lock-in zone. Panels (b) and (c) are enlarged portions of panel (a). Black lines show individual density data, while the red lines are 1 cm running means. Blue and orange lines represent the boundaries of the LIZ estimated from the gas compositions (between two vertical blue lines) and the critical porosity thresholds (between two orange vertical lines), respectively (see Sect. 3.4).
At Styx Glacier, the shallowest depth where the running mean of total porosity with a 1 cm thick window reaches below 0.1 is 48.1 m (Fig. 6a and b). It is approximately 4.3 m shallower than the LID of 52.4 m defined by the modeled firn air δ15N−N2 profile. Meanwhile, the deepest point, where the running mean (with a 1 cm thick window) exceeds 0.1, is at 63.7 m (Fig. 6a and c), which is shallower than the zCOD of 64.8 m defined by the deepest successful firn pumping depth. Although the LID and zCOD from the density data are different from those defined by firn air data, the thickness of LIZ from density data (between the two orange lines in Fig. 6a) is comparable to that from firn air analysis (between two blue lines in Fig. 6) (15.6 vs. 12.4 m). The offsets of the LIZ about 1–4 m between those from total porosity and the firn air measurement may be due to, for example, small calibration offsets in the density data set or the fact that actual critical porosity may be variable and depend on the study site or on horizontal snow density variations and the horizontal extent of diffusion-impeding layers. The similarity in the LIZ thicknesses from the two methods supports the idea that the large variations in density can increase the LIZ thickness by shallowing LID and/or deepening the zCOD. The thick LIZ eventually permits storing old firn air at Styx (Table 1). Usually, the LIZ thickness increases with a snow accumulation rate (Witrant et al., 2012), presumably because at high-accumulation sites density variability in the lock-in zone tends to increase (Hörhold et al., 2011). Refrozen melt layers may also act as high density, diffusion-impeding layers allowing for older firn air to be sampled as observed on Devon Island (Witrant et al., 2012). We demonstrate here that the snow density variability is an important factor in determining the firn air age. We suggest that sites with higher density variations at the LIZ have a high possibility of a thick LIZ and therefore old firn air, even in warm, relatively high-precipitation coastal climates.
To quantitatively compare density variability of Styx snow with that at other glacier sites, we may use the standard deviation of densities (σρ) near the mean air-isolation density (Hörhold et al., 2011; Martinerie et al., 1992). The mean density at the mean air-isolation depth (ρcrit) can be related to mean annual temperature (T in kelvin) using the following equation, which is empirically obtained from air content measurements (Martinerie et al., 1992):
where ρice is the density of bubble-free pure ice.
Table 2Comparison of standard deviation of density (σρ) at critical density (ρcrit). For data from all other sites, except Styx, refer to Hörhold et al. (2011).
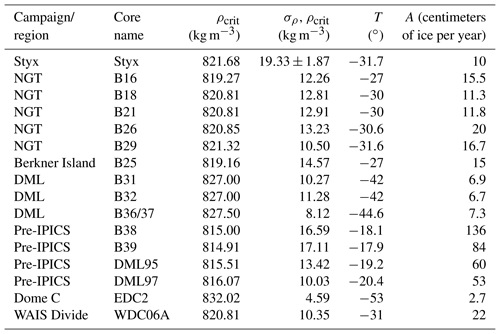
Although this equation cannot provide exact ρcrit, we can take advantage by estimating the density at LIZ without gas chemistry data (Hörhold et al., 2011). We note that Martinerie et al. (1994) suggested slightly different coefficients for the equation based on a different set of data; however, the results do not significantly change our conclusions. We also note that Bréant et al. (2017) used an equation relating ice density at LID to snow accumulation rate; however, we prefer to use the relation of temperature–ice density at LIZ by Martinerie et al. (1992) because the latter is more relevant to the ice density at LIZ. Using the Styx high-resolution X-ray density data at the depth interval of 43.13–66.97 m, we calculated the standard deviation of densities (σρ). For each σρ, we used 1000 density data points (Fig. 7) as Hörhold et al. (2011) did for the other sites listed in Table 2. At Styx, ρcrit is 821.68 kg m−3 according to Eq. (4), and the standard deviation of densities at ρcrit (σρ, ρcrit) is 19.33±1.87 kg m−3, which is greater than those at the other previously studied sites (Hörhold et al., 2011; Fig. 7, Table 2). The high σρ and ρcrit at Styx likely facilitate the thick LIZ and old firn air.
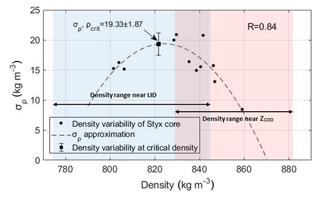
Figure 7Density variability calculated from 1000 depth points and their average density. The standard deviation at the critical density (821.68 kg m−3) calculated from the approximate second-order polynomial (R=0.84) is 19.33±1.87 kg m−3. The blue and red areas are the density ranges near the LID (52.38–52.48 m) and the zCOD (64.91–65.01 m), respectively.
A high-density (low-density) layer at the surface may become a low-density (high-density) layer (Freitag et al., 2004; Fujita et al., 2009) at density of 600–650 kg m−3, which occurs at shallower depths than LIZ (Hörhold et al., 2011). Thus, vertical snow layering at the surface may not directly give information about density variability at LIZ (Hörhold et al., 2011). However, conditions for snow layering at the surface still may give us clues on the density variability at LIZ. The conditions may include redistribution of snow by wind and formation of wind and/or radiation crusts (Martinerie et al., 1992; Hörhold et al., 2011). To test the possibility of seasonal causes, we analyzed stable isotopes of surface snow (δ18O) because the surface δ18O generally follows seasonal variation (depleted in winter and enriched in summer). Figure 2e and f show the stable isotope profiles of snow (δ18O) at Styx Glacier, which are ∼100 m apart; one is from a snow pit made in 2014 and the other is from the main ice core drilled in 2014. The δ18O profiles commonly show cycles with intervals of ∼40 cm yr−1, given that local maxima of δ18O indicate summer, and minima winter layers. Meanwhile, the repetition of the density layers has 20 cycles (high- and low-density layer pairs) in the top 180 cm at the snow pit (Fig. 2b). Using a snow accumulation rate of ∼40 cm yr−1 in recent years, the density layers have four to five cycles per year, indicating that the formation of snow density layers is mainly controlled by nonseasonal factors.
A blizzard occurred during the ice coring campaign in December of 2014. We observed that the blizzard strongly reworked the surface snow. The automatic weather system (AWS) installed within 10 m from the borehole site shows that blizzard events (wind speed >15 m s−1) took place on 29 December in 2015 and 23 May, 26 June, 17 August, and 7 September in 2016 (Fig. S1 in the Supplement). The number of blizzard events in a year is similar to the mean density layer cycle of four to five per year. Although Blizzards occur more frequently in winter, the frequency of five per year is comparable to the number of the density layer cycles of four to five per year. During the blizzard events, westerly wind prevailed, and snow particles may have been redeposited with a sorted size distribution (large grains in the bottom and small grains on the top) similar to winnowing seen in sedimentary records (Sepp Kipfstuhl, personal communication, 2016). Between the blizzards, the solar radiation and temperature gradient may have facilitated the diagenesis of the snow layers (Alley, 1988; Fegyveresi et al., 2018). During the diagenesis processes, fine and coarse flake layers may form high-density and low-density layers, respectively. In summary, blizzard events may have played a major role in forming snow density layers
About 93-year-old firn air (effective CO2 age) was found at Styx Glacier, East Antarctica, located near the Ross Sea coast. This is of great scientific interest because such old firn air is commonly only found in the inland sites such as the South Pole and Megadunes. The thickness of Styx LIZ is relatively greater than that at other sites where snow accumulation and temperature are similar. The thicker LIZ made the Styx firn layer preserve old firn air because the age of stagnant firn air rapidly increases with depth in the LIZ as air exchange with the atmosphere has stopped. We hypothesized that the high snow density variations in the LIZ of Styx Glacier made the thick LIZ and old firn air. To test the hypothesis, we conducted high-resolution X-ray density measurements. We argue that the thick LIZ is related to the high density variations at Styx Glacier. We also examined why high snow density variability developed at the Styx site. The effect of strong wind (e.g., blizzards) may facilitate the density layer formation. It is likely that old firn air (>55 years) can be found in areas where climatological conditions are favorable for high snow density variations at LIZ even when the sites are located near the coast. We may take advantage by sampling and transportation from the coastal sites because logistics is easier for those sites. Theoretically, the oldest firn air should be available at a site that has both strong layering and a low accumulation rate. Older firn air, perhaps as old as 150 years, may still be found under such suitable conditions on the Antarctic continent.
The firn air composition data will be available at the NOAA Paleoclimatology dataset portal in the near future and can be accessed in the meantime by contacting the corresponding author. Other ice chemistry and density data are available upon request as well.
The supplement related to this article is available online at: https://doi.org/10.5194/tc-13-2407-2019-supplement.
JA and YJ designed and led the research; YJ and CB performed firn air diffusion modeling; SBH, HL, SH, JY, YI, AH, YH, SJJ, PT, TC, and SH produced analytical data; CB, SK, JA, and all the other co-authors participated in data interpretation.
The authors declare that they have no conflict of interest.
We thank Jeff Severinghaus and Ross Beaudette at Scripps Institution of Oceanography for accurate δ15N−N2 analysis, and Jacob Schwander at University of Bern for kind advice in constructing the SNU firn air sampling device. We also thank Mauro Rubino and the anonymous reviewer for their constructive comments.
This research has been supported by the Korea Polar Research Institute (grant no. PE 18040) and the National Research Foundation of Korea (grant no. NRF-2018R1A2B3003256).
This paper was edited by Joel Savarino and reviewed by Mauro Rubino and one anonymous referee.
Alley, R. B.: Concerning the Deposition and Diagenesis of Strata in Polar Firn, J. Glaciol., 34, 283–290, https://doi.org/10.3189/s0022143000007024, 1988.
Aydin, M., Montzka, S. A., Battle, M. O., Williams, M. B., De Bruyn, W. J., Butler, J. H., Verhulst, K. R., Tatum, C., Gun, B. K., Plotkin, D. A., Hall, B. D., and Saltzman, E. S.: Post-coring entrapment of modern air in some shallow ice cores collected near the firn-ice transition: evidence from CFC-12 measurements in Antarctic firn air and ice cores, Atmos. Chem. Phys., 10, 5135–5144, https://doi.org/10.5194/acp-10-5135-2010, 2010.
Battle, M., Bender, M., Sowers, T., Tans, P. P., Butler, J. H., Elkins, J. W., Ellis, J. T., Conway, T., Zhang, N., Lang, P., and Clarke, A. D.: Atmospheric gas concentrations over the past century measured in air from firn at the South Pole, Nature, 383, 231–235, 1996.
Battle, M. O., Severinghaus, J. P., Sofen, E. D., Plotkin, D., Orsi, A. J., Aydin, M., Montzka, S. A., Sowers, T., and Tans, P. P.: Controls on the movement and composition of firn air at the West Antarctic Ice Sheet Divide, Atmos. Chem. Phys., 11, 11007–11021, https://doi.org/10.5194/acp-11-11007-2011, 2011.
Blunier, T. and Schwander, J.: Gas enclosure in ice: age difference and fractionation, in: Physics of Ice Core Records, edited by: Hondoh, T., Hokkaido University Press, Sapporo, Japan, 307–326, 2000.
Bréant, C., Martinerie, P., Orsi, A., Arnaud, L., and Landais, A.: Modelling firn thickness evolution during the last deglaciation: constraints on sensitivity to temperature and impurities, Clim. Past, 13, 833–853, https://doi.org/10.5194/cp-13-833-2017, 2017.
Buizert, C.: The influence of firn air transport processes and radiocarbon production on gas records from polar firn and ice, PhD, Faculty of Science, University of Copenhagen, Denmark, Copenhagen, 175 pp., 2012.
Buizert, C. and Severinghaus, J. P.: Dispersion in deep polar firn driven by synoptic-scale surface pressure variability, The Cryosphere, 10, 2099–2111, https://doi.org/10.5194/tc-10-2099-2016, 2016.
Buizert, C., Martinerie, P., Petrenko, V. V., Severinghaus, J. P., Trudinger, C. M., Witrant, E., Rosen, J. L., Orsi, A. J., Rubino, M., Etheridge, D. M., Steele, L. P., Hogan, C., Laube, J. C., Sturges, W. T., Levchenko, V. A., Smith, A. M., Levin, I., Conway, T. J., Dlugokencky, E. J., Lang, P. M., Kawamura, K., Jenk, T. M., White, J. W. C., Sowers, T., Schwander, J., and Blunier, T.: Gas transport in firn: multiple-tracer characterisation and model intercomparison for NEEM, Northern Greenland, Atmos. Chem. Phys., 12, 4259–4277, https://doi.org/10.5194/acp-12-4259-2012, 2012.
Craig, H., Horibe, Y., and Sowers, T.: Gravitational separation of gases and isotopes in polar ice caps, Science, 242, 1675–1678, 1988.
Dlugokencky, E. J., Myers, R. C., Lang, P. M., Masarie, K. A., Crotwell, A. M., Thoning, K. W., Hall, B. D., Elkins, J. W., and Steele, L. P.: Conversion of NOAA atmospheric dry air CH4 mole fractions to a gravimetrically prepared standard scale, J. Geophys. Res., 110, D18306, https://doi.org/10.1029/2005JD006035, 2005.
Etheridge, D. M., Pearman, G. I., and Fraser, P. J.: Changes in tropospheric methane between 1841 and 1978 from a high accumulation-rate Antarctic ice core, Tellus B, 44, 282–294, https://doi.org/10.1034/j.1600-0889.1992.t01-3-00006.x, 1992.
Etheridge, D. M., Steele, L. P., Langenfelds, R. L., Francey, R. J., Barnola, J. M., and Morgan, V. I.: Natural and anthropogenic changes in atmospheric CO2 over the last 1000 years from air in Antarctic ice and firn, J. Geophys. Res., 101, 4115–4128, https://doi.org/10.1029/95jd03410, 1996.
Fegyveresi, J. M., Alley, R. B., Muto, A., Orsi, A. J., and Spencer, M. K.: Surface formation, preservation, and history of low-porosity crusts at the WAIS Divide site, West Antarctica, The Cryosphere, 12, 325-341, https://doi.org/10.5194/tc-12-325-2018, 2018.
Fourteau, K., Faïn, X., Martinerie, P., Landais, A., Ekaykin, A. A., Lipenkov, V. Ya., and Chappellaz, J.: Analytical constraints on layered gas trapping and smoothing of atmospheric variability in ice under low-accumulation conditions, Clim. Past, 13, 1815–1830, https://doi.org/10.5194/cp-13-1815-2017, 2017.
Freitag, J., Wilhelms, F., and Kipfstuhl, S.: Microstructure dependent densification of polar firn derived from X-ray microtomography, J. Glaciol., 50, 243–250, 2004.
Fujita, S., Okuyama, J., Hori, A., and Hondoh, T.: Metamorphism of stratified firn at dome fuji, antarctica: A mechanism for local insolation modulation of gas transport conditions during bubble close off, J. Geophys. Res., 114, F03023, https://doi.org/10.1029/2008JF001143, 2009.
Goujon, C., Barnola, J. M., and Ritz, C.: Modeling the densification of polar firn including heat diffusion: Application to closeoff characteristics and gas isotopic fractionation for Antarctica and Greenland sites, J. Geophys. Res.-Atmos., 108, 4792, https://doi.org/10.1029/2002JD003319, 2003.
Gregory, S. A., Albert, M. R., and Baker, I.: Impact of physical properties and accumulation rate on pore close-off in layered firn, The Cryosphere, 8, 91–105, https://doi.org/10.5194/tc-8-91-2014, 2014.
Han, Y., Jun, S. J., Miyahara, M., Lee, H.-G., Ahn, J., Chung, J. W., Hur, S. D., and Hong, S. B.: Shallow ice-core drilling on Styx glacier, northern Victoria Land, Antarctica in the 2014–2015 summer, Journal of the Geological Society of Korea, 51, 343–355, 2015
Hörhold, M. W., Kipfstuhl, S., Wilhelms, F., Freitag, J., and Frenzel, A.: The densification of layered polar firn, J. Geophys. Res.-Earth, 116, F01001, https://doi.org/10.1029/2009jf001630, 2011.
Hori, A., Tayuki, K., Narita, H., Hondoh, T., Fujita, S., Kameda, T., Shoji, H., Azuma, N., Kamiyama, K., Fujii, Y., Motoyama, H., and Watanabe, O.: A detailed density profile of the Dome Fuji (Antarctica) shallow ice core by X-ray transmission method, Ann. Glaciol., 29, 211–214, https://doi.org/10.3189/172756499781821157, 1999.
Johnsen, S. J., Clausen, H. B., Cuffey, K. M., Hoffmann, G., Schwander, J., and Creyts, T.: Diffusion of stable isotopes in polar firn and ice: the isotope effect in firn diffusion, in: Physics of Ice Core Records, edited by: Hondoh, T., vol. 159, 121–140, Hokkaido University Press, Sapporo, Japan, 2000.
Kawamura, K., Severinghaus, J. P., Ishidoya, S., Sugawara, S., Hashida, G., Motoyama, H., Fujii, Y., Aoki, S., and Nakazawa, T.: Convective mixing of air in firn at four polar sites, Earth Planet. Sc. Lett., 244, 672–282, 2006.
Kawamura, K., Severinghaus, J. P., Albert, M. R., Courville, Z. R., Fahnestock, M. A., Scambos, T., Shields, E., and Shuman, C. A.: Kinetic fractionation of gases by deep air convection in polar firn, Atmos. Chem. Phys., 13, 11141–11155, https://doi.org/10.5194/acp-13-11141-2013, 2013.
Landais, A., Barnola, J. M., Kawamura, K., Caillon, N., Delmotte, M., Van Ommen, T., Dreyfus, G., Jouzel, J., Masson-Delmotte, V., Minster, B., Freitag, J., Leuenberger, M., Schwander, J., Huber, C., Etheridge, D., and Morgan, V.: Firn-air δ15N in modern polar sites and glacial–interglacial ice: a model-data mismatch during glacial periods in Antarctica?, Quaternary Sci. Rev., 25, 49–62, https://doi.org/10.1016/j.quascirev.2005.06.007, 2006.
Laube, J. C., Hogan, C., Newland, M. J., Mani, F. S., Fraser, P. J., Brenninkmeijer, C. A. M., Martinerie, P., Oram, D. E., Röckmann, T., Schwander, J., Witrant, E., Mills, G. P., Reeves, C. E., and Sturges, W. T.: Distributions, long term trends and emissions of four perfluorocarbons in remote parts of the atmosphere and firn air, Atmos. Chem. Phys., 12, 4081–4090, https://doi.org/10.5194/acp-12-4081-2012, 2012.
MacFarling Meure, C., Etheridge, D., Trudinger, C., Steele, P., Langenfelds, R., van Ommen, T., Smith, A., and Elkins, J.: Law Dome CO2, CH4 and N2O ice core records extended to 2000 years BP, Geophys. Res. Lett., 33, L14810, https://doi.org/10.1029/2006GL026152, 2006.
Martinerie, P., Raynaud, D., Etheridge, D. M., Barnola, J. M., and Mazaudier, D.: Physical and Climatic Parameters which Influence the Air Content in Polar Ice, Earth Planet. Sc. Lett., 112, 1–13, https://doi.org/10.1016/0012-821X(92)90002-D, 1992.
Martinerie, P., Lipenkov, V. Y., Raynaud, D., Chappellaz, J., Barkov, N. I., and Lorius, C.: Air content paleo record in the Vostok ice core (Antarctica): A mixed record of climatic and glaciological parameters, J. Geophys. Res., 99, 10565–10576, https://doi.org/10.1029/93JD03223, 1994.
Mitchell, L. E., Buizert, C., Brook, E. J., Breton, D. J., Fegyveresi, J., Baggenstos, D., Orsi, A., Severinghaus, J., Alley, R. B., Albert, M., Rhodes, R. H., McConnell, J. R., Sigl, M., Maselli, O., Gregory, S., and Ahn, J.: Observing and modeling the influence of layering on bubble trapping in polar firn, J. Geophys. Res.-Atmos., 120, 2558–2574, https://doi.org/10.1002/2014JD022766, 2015.
Petit, J. R., Jouzel, J., Raynnaud, D., Barkov, N. I., Barnola, J.-M., Basile, I., Bender, M., Chappellaz, J., Davis, M., Delaygue, G., Delmotte, M., Kotlyakov, V. M., Legrand, M., Lipenkov, V. Y., Lorius, C., Pepin, L., Ritz, C., Saltzman, E., and Stievenard, M.: Climate and atmospheric history of the past 420 000 years from the Vostok ice core, Antarctica, Nature, 399, 429–436, 1999.
Proksch, M., Rutter, N., Fierz, C., and Schneebeli, M.: Intercomparison of snow density measurements: bias, precision, and vertical resolution, The Cryosphere, 10, 371–384, https://doi.org/10.5194/tc-10-371-2016, 2016.
Rhodes, R. H., Fain, X., Stowasser, C., Blunier, T., Chappellaz, J., McConnell, J. R., Romanini, D., Mitchell, L. E., and Brook, E. J.: Continuous methane measurements from a late Holocene Greenland ice core: atmospheric and in-situ signals, Earth Planet. Sc. Lett., 368, 9–19, 2013.
Rhodes, R. H., Faïn, X., Brook, E. J., McConnell, J. R., Maselli, O. J., Sigl, M., Edwards, J., Buizert, C., Blunier, T., Chappellaz, J., and Freitag, J.: Local artifacts in ice core methane records caused by layered bubble trapping and in situ production: a multi-site investigation, Clim. Past, 12, 1061–1077, https://doi.org/10.5194/cp-12-1061-2016, 2016.
Rubino, M., Etheridge, D. M., Thornton, D. P., Howden, R., Allison, C. E., Francey, R. J., Langenfelds, R. L., Steele, L. P., Trudinger, C. M., Spencer, D. A., Curran, M. A. J., van Ommen, T. D., and Smith, A. M.: Revised records of atmospheric trace gases CO2, CH4, N2O, and δ13C−CO2 over the last 2000 years from Law Dome, Antarctica, Earth Syst. Sci. Data, 11, 473–492, https://doi.org/10.5194/essd-11-473-2019, 2019.
Schaller, C. F., Freitag, J., and Eisen, O.: Critical porosity of gas enclosure in polar firn independent of climate, Clim. Past, 13, 1685–1693, https://doi.org/10.5194/cp-13-1685-2017, 2017.
Schwander, J.: The transformation of snow to ice and the occlusion of gases, Environ. Rec. Glaciers Ice Sheets, 8, 53–67, 1989.
Schwander, J., Barnola, J.-M., Andrié, C., Leuenberger, M., Ludin, A., Raynaud, D., and Stauffer, B.: The age of the air in the firn and the ice at Summit, Greenland, J. Geophys. Res.-Atmos., 98, 2831–2838, https://doi.org/10.1029/92jd02383, 1993.
Severinghaus, J. P., Grachev, A., and Battle, M.: Thermal fractionation of air in polar firn by seasonal temperature gradients, Geochem. Geophy. Geosy., 2, 1048, https://doi.org/10.1029/2000GC000146, 2001.
Severinghaus, J. P., Albert, M. R., Courville, Z. R., Fahnestock, M. A., Kawamura, K., Montzka, S. A., Mühle, J., Scambos, T. A., Shields, E., Shuman, C. A., Suwa, M., Tans, P., and Weiss, R. F.: Deep air convection in the firn at a zero-accumulation site, central Antarctica, Earth Planet. Sc. Lett., 293, 359–367, https://doi.org/10.1016/j.epsl.2010.03.003, 2010.
Sowers, T., Bender, M., Raynaud, D., and Korotkevich, Y. S.: Delta n-15 of n2 in air trapped in polar ice – a tracer of gas-transport in the firn and a possible constraint on ice age-gas age-differences, J. Geophys. Res.-Atmos., 97, 15683–15697, 1992.
Stauffer, B., Schwander, J., and Oeschger, H.: Enclosure of air during metamorphosis of dry firn to ice, Ann. Glaciol., 6, 108–112, https://doi.org/10.3189/1985AoG6-1-108-112, 1985.
Trudinger, C. M., Enting, I. G., Etheridge, D. M., Francey, R. J., Levchenko, V. A., Steele, L. P., Raynaud, D., and Arnaud, L.: Modeling air movement and bubble trapping in firn, J. Geophys. Res.-Atmos., 102, 6747–6763, https://doi.org/10.1029/96JD03382, 1997.
Trudinger, C. M., Enting, I. G., Rayner, P. J., and Francey, R. J.: Kalman filter analysis of ice core data 2. Double deconvolution of CO2 and δ13C measurements, J. Geophys. Res., 107, 4423, https://doi.org/10.1029/2001JD001112, 2002.
Trudinger, C. M., Enting, I. G., Rayner, P. J., Etheridge, D. M., Buizert, C., Rubino, M., Krummel, P. B., and Blunier, T.: How well do different tracers constrain the firn diffusivity profile?, Atmos. Chem. Phys., 13, 1485–1510, https://doi.org/10.5194/acp-13-1485-2013, 2013.
Witrant, E., Martinerie, P., Hogan, C., Laube, J. C., Kawamura, K., Capron, E., Montzka, S. A., Dlugokencky, E. J., Etheridge, D., Blunier, T., and Sturges, W. T.: A new multi-gas constrained model of trace gas non-homogeneous transport in firn: evaluation and behaviour at eleven polar sites, Atmos. Chem. Phys., 12, 11465–11483, https://doi.org/10.5194/acp-12-11465-2012, 2012.
Yang, J. W.: Paleoclimate reconstructions from greenhouse gas and borehole temperature of polar ice cores, and study on the origin of greenhouse gas in permafrost ice wedges, PhD thesis, Department of Earth and Environmental Sciences, Seoul National University, Seoul, Republic of Korea, 188 pp., 2019.
Yang, J.-W., Ahn, J., Brook, E. J., and Ryu, Y.: Atmospheric methane control mechanisms during the early Holocene, Clim. Past, 13, 1227–1242, https://doi.org/10.5194/cp-13-1227-2017, 2017.
Yang, J. W., Han, Y., Orsi, A. J., Kim, S. J., Han, H., Ryu, Y., Jang, Y., Moon, J., Choi, T., Hur, S. D., and Ahn, J.: Surface temperature in twentieth century at the Styx Glacier, northern Victoria Land, Antarctica, from borehole thermometry, Geophys. Res. Lett., 45, 9834–9842, https://doi.org/10.1029/2018GL078770, 2018.