the Creative Commons Attribution 4.0 License.
the Creative Commons Attribution 4.0 License.
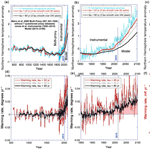
Climate change and the global pattern of moraine-dammed glacial lake outburst floods
Stephan Harrison
Jeffrey S. Kargel
Christian Huggel
John Reynolds
Dan H. Shugar
Richard A. Betts
Adam Emmer
Neil Glasser
Umesh K. Haritashya
Jan Klimeš
Liam Reinhardt
Yvonne Schaub
Andy Wiltshire
Dhananjay Regmi
Vít Vilímek
Despite recent research identifying a clear anthropogenic impact on glacier recession, the effect of recent climate change on glacier-related hazards is at present unclear. Here we present the first global spatio-temporal assessment of glacial lake outburst floods (GLOFs) focusing explicitly on lake drainage following moraine dam failure. These floods occur as mountain glaciers recede and downwaste. GLOFs can have an enormous impact on downstream communities and infrastructure. Our assessment of GLOFs associated with the rapid drainage of moraine-dammed lakes provides insights into the historical trends of GLOFs and their distributions under current and future global climate change. We observe a clear global increase in GLOF frequency and their regularity around 1930, which likely represents a lagged response to post-Little Ice Age warming. Notably, we also show that GLOF frequency and regularity – rather unexpectedly – have declined in recent decades even during a time of rapid glacier recession. Although previous studies have suggested that GLOFs will increase in response to climate warming and glacier recession, our global results demonstrate that this has not yet clearly happened. From an assessment of the timing of climate forcing, lag times in glacier recession, lake formation and moraine-dam failure, we predict increased GLOF frequencies during the next decades and into the 22nd century.
- Article
(3963 KB) - Full-text XML
-
Supplement
(989 KB) - BibTeX
- EndNote
There is increasing scientific and policy interest in detecting climate change impacts and assessing the extent to which these can be attributable to anthropogenic or natural causes. As a result, recent research demonstrating an anthropogenic fingerprint on a significant proportion of recent global glacier recession is an important step forward (Marzeion et al., 2014). The focus can now shift to glacier hazards but the complex nature of glacier–climate interactions (Roe et al., 2017) and their influence on hazards makes this a challenging task (Shugar et al., 2017).
Mountain glaciers have continued to recede (Kargel et al., 2014; Cramer et al., 2014) and thin from their late Holocene (Little Ice Age, LIA) positions and, in many cases, the rate of recession and thinning has increased over recent decades largely as a consequence of global warming (Marzeion et al., 2014). Thinning, flow stagnation and recession of glacier tongues have resulted in the formation of moraine-dammed lakes (Richardson and Reynolds, 2000). These moraines, some of which contain a melting ice core, are built from rock debris transported by glaciers. When they fail, large volumes of stored water can be released, producing glacial lake outburst floods (GLOFs). These floods have caused thousands of fatalities and severe impacts on downstream communities, infrastructure and long-term economic development (Mool et al., 2011; Riaz et al., 2014; Carrivick and Tweed, 2016).
Although much research has been carried out on the nature and characteristics of GLOFs and hazardous lakes from many of the world's mountain regions (e.g. Lliboutry et al., 1977; Evans, 1987; O'Connor et al., 2001; Huggel et al., 2002; Bajracharya and Mool, 2009; Ives et al., 2010; Iribarren et al., 2014; Lamsal et al., 2014; Vilímek et al., 2014; Westoby et al., 2014; Perov et al., 2017), there are significant gaps in our knowledge of these phenomena at the global scale and concerning their relationship to anthropogenic climate change. Detecting changes in the magnitude, timing and frequency of glacier-related hazards over time and assessing whether changes can be related to climate forcing and glacier dynamical responses is also of considerable scientific and economic interest (Oerlemans, 2005; Stone et al., 2013). Multiple case studies are insufficient to achieve a better understanding of the mechanisms leading to GLOF initiation so a more comprehensive understanding of the global frequency and timing of GLOFs is necessary. Testing such relationships at a global scale is also an important step toward assessment of the sensitivity of geomorphological systems to climate change.
Despite numerous inventories of GLOFs at regional scales (see Emmer et al., 2016), no global database has been created which focuses specifically on GLOFs relating to the failure of moraine dams. A global database is required to place GLOFs in their wider climatic context (Richardson and Reynolds, 2000; Mool et al., 2011). This means that we are unable to answer some important questions concerning their historic behaviour and therefore the changing magnitude and frequency of GLOFs globally through time, and their likely evolution under future global climate change. This latter point is made even more difficult by the lack of long-term climate data from many mountain regions. Given the size and impacts of GLOFs in many mountain regions, better understanding their links to present and future climate change is of great interest to national and regional governments, infrastructure developers and other stakeholders. We argue that glacier hazard research needs to be increasingly seen through the lens of change adaptation.
These issues and knowledge gaps can be addressed via a systematic, uniform database of GLOFs. Here we have compiled an unprecedented global GLOF inventory related to the failure of moraine dams. We discuss the problems involved in developing a robust attribution argument concerning GLOFs and climate change. This inventory covers only the subset of GLOFs that are linked to overtopping or failure of moraine dams. Our focus on moraine dams is motivated by (1) this type of event leaving clear diagnostic evidence of moraine-dam failures in the form of breached end moraines and lake basins, whereas ice-dammed lake failures commonly do not leave such clear and lasting geomorphological evidence and (2) the conventional hypothetical link between climate change, glacier response, moraine-dammed lake formation and GLOF production being more straightforward compared to the range of processes driving GLOFs from ice- and bedrock-dammed lakes.
Such GLOF events are often triggered by ice and rockfalls, rockslides or moraine failures into lakes, creating seiche or displacement waves, but also by heavy precipitation or ice melt/snowmelt events (Richardson and Reynolds, 2000). While climate change plays a dominant role in the recession of glaciers, downwasting glacier surfaces debuttress valley rock walls, leading to catastrophic failure in the form of rock avalanches or other types of landslides (Ballantyne, 2002; Shugar and Clague, 2011; Vilímek et al., 2014). Other climatically induced triggers of moraine dam failures include increased permafrost and glacier temperatures leading to failure of ice and rock masses into lakes and the melting of ice cores in moraine dams, which leads to moraine failure and lake drainage.
Attribution of climate change impacts is an emerging research field and no attribution studies on GLOFs are available so far. Even for glaciers only very few attribution studies have been published to date (Marzeion et al., 2014; Roe et al., 2017). Follow-up studies from the IPCC 5th Assessment Report (Cramer et al., 2014) proposed a methodological procedure to attribute impacts to climate change (Stone et al., 2013). Based on that, a methodologically sound detection and attribution study needs first to formulate a hypothesis of potential impacts of climate change. In our case physical process understanding supports the association between climate change and GLOFs associated with moraine-dam failure by climate warming, resulting in glacier recession and glacial lake formation and evolution behind moraine dams which become unstable and fail catastrophically. The next step requires a climate trend to be detected, followed by the identification of the baseline behaviour of the system in the absence of climate change. The difficulty of identifying the baseline behaviour is related to several factors. The first is the existence of confounding factors, both natural and human related. For instance, the frequency of GLOFs from moraine dams also depends on factors such as the stability of the dam, including dam geometry and material or mitigation measures such as artificial lowering of the lake level (Portocarreo-Rodriguez and the Engility Corporation, 2014). Second, there are few long-term palaeo-GLOF records with which to assess baseline behaviour. Eventually, attribution includes the detection of an observed change that is consistent with the response to the climate trend, in our case a change in GLOF occurrence and the evaluation of the contribution of climate change to the observed change in relation to confounding factors. Our chief observational result is that there is an upsurge in GLOF frequency starting around 1930 and then a decline following roughly 1975 and persisting for decades (see also Carrivick and Tweed, 2014). At face value, when comparing this with the climate records, there seems to be no relationship between global GLOF frequency and concurrent climatic fluctuations, and a regional breakdown offers no solution; for example, strong climatic global (or Northern Hemisphere) warming during the period of declining GLOF frequency after 1975 appears to be counterintuitive. A simplistic inference would be that climate change does not influence GLOF incidence, but we reject this given our understanding of the physical drivers of glacier recession, lake development and drainage mechanisms. Although we know that GLOFs involve a complex set of dynamics, one of the important dynamical changes affecting GLOFs is the formation and growth of glacial lakes, and we know that there must be a relationship here to climatic warming. GLOF triggers also commonly involve extreme weather, such as extreme heat and extreme precipitation, which are intuitively linked to climate change as well, even if the attribution experiments have not yet been carried out. We thus have to dig deeper to see how GLOF frequency may be connected to climate change. The point arises that the conditions needed for a GLOF involve a long period of lake formation and growth, such that past climate changes are involved. In the Methods section we produce a model whereby the history of one climate variable and its time derivative – Northern Hemisphere mean temperature and warming rate – are linked to the GLOF record.
We produced a database of GLOFs developed from a collation of regional inventories and reviews (e.g. GAPHAZ1, WGMS2and GLACIORISK3 databases and the GLOF Database provided under ICL database of glacier and permafrost disasters from the University of Oslo, Reynolds and Richardson, 2000; RGSL, 1997, 2002), regional overviews and reviews (e.g. Clague et al., 1985; Xu, 1987; Costa and Schuster, 1988; Reynolds, 1992; Ding and Liu, 1992; Clague and Evans, 2000; O'Connor et al., 2001; Zapata, 2002; Raymond et al., 2003; Jiang et al., 2004; Carey, 2005; Osti and Egashira, 2009; Narama et al., 2010; Ives et al., 2010; Wang et al., 2011; Carey et al., 2012; Mergili and Schneider, 2011; Fujita et al., 2012; Iribarren et al., 2014, Emmer, 2017) and case studies of individual GLOFs (e.g. Kershaw et al., 2005; Harrison et al., 2006; Worni et al., 2012). A complete list is available in the Supplement. The GLOF database was developed from a collation of regional inventories and reviews (see Supplement). Only GLOFs that could be dated to the year and to moraine failure were included. Past temperature trends from the glacier regions of interest were extracted from three independent global temperature reconstructions (CRUTEM4.2, Jones et al., 2012, NOAA NCDC (National Oceanic and Atmospheric Administration – National Climatic Data Center), Smith et al., 2008, and NASA GISTEMP (Goddard's Institute Surface Temperature Analysis), Hansen et al., 2010). These data sets provided temperature anomaly data relative to a modern baseline beginning in 1850 for CRUTEM4.2 and 1880 for NOAA NCDC and NASA GISTEMP.
2.1 Test of direct linkage between GLOF rate and climate change
We concentrate exclusively on the subset of GLOFs associated with the failure of moraine-dammed lakes as these are a major hazard in many mountain regions but also represent the best candidates of outburst floods for attribution to climate change. We differentiate these from other glacially sourced outburst floods, such as those resulting from the failure of an ice dam (Walder and Costa, 1996; Tweed and Russell, 1999; Roberts et al., 2003), dam overflow, volcanically triggered jökulhlaups (Carrivick et al., 2004; Russell et al., 2010; Dunning et al., 2013) or the sudden release of water from englacial or subglacial reservoirs (Korup and Tweed, 2007).
The period over which climate data are available is dependent on the region but starts in 1850 in CRUTEM4.2 and 1880 in NOAA NCDC and NASA GISTEMP. The resolution of the data is generally 5∘; however, NASA GISTEMP is provided at 1∘ resolution but it should be noted this does not imply there are more observational data in this analysis. For each region, we extract all grid points that contain a glacier as defined in the World Glacier Inventory – Extended Format (WGI-XF). With the exception of the European Alps no data set contains a complete continuous record for the period 1900–2012. We therefore take all available data points to form time series for each data set and derive a mean linear trend for the 1990–2012 period. Given large uncertainties and data gaps no attempt is made to statistically test these trends. The trends presented here are therefore considered illustrative of past changes in temperature for these regions.
Wavelet analysis of GLOF incidence
Wavelets are a commonly used tool for analysing non-stationary time series because they allow the signal to be decomposed into both time and frequency (e.g. Lane, 2007). Here, we follow the methodology of Shugar et al. (2010), although we use the Daubechies (db1) continuous wavelet. The wavelet power shown here has been tested for significance at 95 % confidence limits, and a cone of influence is applied to reduce edge effects. We follow Lane (2007) in choosing an appropriate number of scales (S=28, see his Eq. 28), which are related to the shape of the cone of influence.
2.2 The Earth's recent climate record smoothed along glacier response timescales: development of the GLOF lag hypothesis
A potentially destructive GLOF may elapse after a glacial lake grows to a volume where a sudden release of glacial lake water can exceed a normal year's peak instantaneous discharge. There are timescales associated with the period between a climatic (or other) perturbation and the occurrence of a GLOF. The following thought experiment demonstrates the concept of the lagging responses of GLOF activity to climate change: an initialized stable condition allows glacier–climate equilibrium, where neither the climate nor the glacier has fluctuated much for some lengthy period and where no other strongly perturbing conditions exist; e.g. there are no significant supraglacial or ice-marginal or moraine-dammed lakes, and a steady state exists in the supply and removal of surface debris. We then impose a perturbation (climatic or other) which favours eventual lake development and growth and eventually a GLOF. We describe two successive time periods which must pass before a significant GLOF can occur, and then a third period before a GLOF actually occurs: lake-inception time (τi), lake growth time (τg) and trigger time (τt). The first two sum to the GLOF response time (τGLOF) as we define it: . The terms are for illustrative purposes: many supraglacial ponds initially go through a lengthy period where they fluctuate and drain annually and thus do not have a chance to grow beyond one season. Furthermore, lakes can grow to a point where limnological processes take over from climate; hence lake growth becomes detached from climate change. Even so, our set of definitions can be used to explain the lagging responses of glacier lakes and GLOFs to climatic history.
A GLOF does not necessarily occur upon climate step change date +τGLOF, which is the timescale over which the metastable system establishes a condition where a significant GLOF could occur. A trigger is needed (e.g. a large ice or rock avalanche into the lake or a moraine collapse as an ice core melts). After a sizeable glacial lake has developed, suitable GLOF triggers may occur with a typical random interval averaging τt, which depends on the topographic setting of the glacier lake, valley-side geology, steepness, moraine dam properties and climate. As a result, τt could range from years to centuries. Furthermore, as a lake usually continues to grow after τGLOF has elapsed, τt can in principle change, probably shortening as the lake lengthens and as the damming moraine degrades. The time elapsing between a climatic perturbation and a GLOF then is the sum of three characteristic sequential periods, .
The lake inception time τi might be approximated by the glacier response time, which has been defined parametrically (Jóhannesson et al., 1989; Bahr et al., 1998) but in general describes a period of adjustment toward a new equilibrium following a perturbation. We take a simple parameterization (Jóhannesson et al., 1989) and equate , where h is the glacier thickness of the tongue near the terminus and b is the annual balance rate magnitude. The glacier response time approximating the lake inception time may be many decades for most temperate valley glaciers, but it can range between a few years and a few centuries. The glacier response time is a climate-change-forgetting timescale. After a few response times have elapsed, a glacier's state and dynamics no longer remember the climate change that induced the response to a new equilibrium. For illustration, we adopt τl=60 years, a value typical of many temperate valley glaciers.
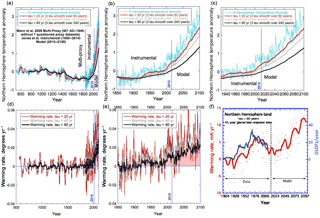
Figure 1Reconciliation of GLOF and climate records. (a) Blue curve: composite record of Northern Hemisphere land surface temperature (merged from multi-proxy data and instrumental records, as described in the main text), plus a model of land surface temperature during the period 2015–2100. Red, grey and black curves: moving historical averages of the blue curve, as described in the text, using τGLOF=20, 40 and 80 years. (b, c) Close-up of the more recent periods covered in (a). (d) Warming rate extracted from the moving historical averages using τGLOF=20, 40 and 80 years. Periods of cooling and warming are shown with blue and red tints, respectively, using the τGLOF=80 years curve. (e) Close-up of (d) to a more recent period. (f) Comparison of a smoothed GLOF frequency curve (red line, GLOFs/year historical moving average) with the moving historical average Northern Hemisphere temperature (black curve) using τGLOF=80 years and shifted +45 years, where the 45-year shift is considered to be reflective of τ, the GLOF trigger timescale. See supplement text for more description and explanation.
A supraglacial pond may drain and redevelop annually (posing no significant GLOF risk), but at some point, if there is a sustained long-term negative mass balance, supraglacial ponds commonly grow, coalesce and form a water body big enough that rapid partial drainage can result in a significant GLOF. That lake growth period is defined here as τg, for which we adopt 20 years, a value typical of many temperate glacier lakes of the 20th century (e.g. Wilson et al., 2018; Emmer et al., 2015) Hence, 80 years for the favoured values. Hence, a significant GLOF may occur at any time from 80 years following a large climatic perturbation: what the GLOF waits on is τt, which could be years or a century. This concept can be extended to the lagging response of a whole population of glaciers following a perturbation in regional climate (Fig. 1).
We distinguish between climate change, which may establish conditions needed for a GLOF to happen, and weather, which sometimes may be involved in a GLOF trigger. GLOF triggers are diverse; e.g. protracted warm summer weather may trigger an ice avalanche into the lake or moraine melt-through, or heavy winter snow may trigger an ice avalanche into the lake.
However, the relevant controlling climate in this example is that of the prior climatic history and the conditioning period defined by τGLOF and the typical trigger interval τt. Hence, τGLOF is closely connected to climate, whereas τt can be connected to weather for certain types of triggers.
The assessment above is for a single step-function climate change. Considering that climate changes continuously and glacier characteristics vary, populations of glaciers must have full distributions of τi, τg and τGLOF. Even while glaciers are still adjusting to any big recent historical climate change, more climate change accrues; glacier and lake dynamics take all that into account, either increasing the likelihood and perhaps size of a GLOF or decreasing or delaying it. Hence, the overall GLOF frequency record cannot be synchronous with climatic fluctuations, and it also should not simply trace past climate change with a time lag; rather, the GLOF frequency record for any large population of glaciers should be definitely but complexly related to the recent climatic history.
The functional dependence on climate history is not known for any glacier or population of glaciers, but to explore the concept of a lagged GLOF response to accrued climate changes, we assert that the integration function will tend to weight recent climatic shifts more strongly than progressively older climatic shifts, the memory of which is gradually lost as the glacier population adjusts. That is, because of glacier dynamics and the responses of a population of glaciers to climatic changes, the population eventually loses memory of sufficiently older climatic changes and adjusts asymptotically toward a new equilibrium. This should be true for any climate-sensitive glacier dynamics (Oerlemans, 2005). Though we do not know the functional form of the glacier responses (either for an individual glacier or a population), we nonetheless wish to illustrate our point while not driving fully quantitative conclusions. We propose that the integration of climate information into ongoing glacier dynamical adjustments occurs with exponentially declining weighting going backward in time from any given year. The exponential time-weighting constant may be similar to τGLOF. We have computed a moving time-average Northern Hemisphere temperature with the weighting of the average specified by an assumed τGLOF= 80 years; the computed moving average pulls data, for any year, over the preceding period of τGLOF; i.e. it includes temperature information up to 240 years prior to any given year. The weighting of earlier years' temperatures within that τGLOF is less than that of later years, according to the exponential. The cut-off at τGLOF is arbitrary and was done for computational expediency, seeing that any climate fluctuation occurring before τGLOF years earlier is inconsequential due to the exponential memory loss.
We combined the Mann et al. (2008) multi-proxy Northern Hemisphere temperature anomaly from 501 AD to 1849, the Jones et al. (2012)4 Northern Hemisphere land instrumental temperature record from 1850 to 2014 and a model of expected warming from 2015 to 2100. It is the recent climate history at each glacier lake or region that is strictly relevant, but lacking such records and needing here to only establish the concept, we settle for the treatment described above involving the Northern Hemisphere temperature anomaly.
The model is a constant 2.7 ∘C century−1 warming; noise was added from a naturally noisy but overall non-trending instrumental record from 1850 to 1899, with some years repeated to append the 2015–2100 period (Fig. 1). The Mann et al. (2008) and Jones et al. (2012) data sets were brought into congruence in 1850. Then we smoothed the composite record and model results using the τGLOF exponentially weighted filter, as described above, where the natural logarithmic “forgetting” timescale τGLOF= 20, 40 or 80 years for three illustrative cases. Smoothing was computed for τGLOF, i.e. 240 years if τGLOF= 80 years. Our favoured value τGLOF= 80 years is based on large Himalayan and other temperate glacier lakes. The shorter response times would likely apply to small glaciers or those occurring in steep valleys.
Regardless of the functional form of the glacier response and lake dynamics, GLOF frequency in any given region or worldwide must lag the climate record. The historically filtered/smoothed temperature record and model incorporating τGLOF= 20, 40 and 80 years is shown in Fig. 1a–c together with the unsmoothed actual record and model temperature series. The temperature anomalies are plotted in panels (a), (b) and (c); and the warming rate in panels (d) and (e). The historically averaged/smoothed temperature record lags fluctuations in the unsmoothed record. The lag is most easily seen where temperatures start to rise rapidly in the 20th and 21st centuries. The high-frequency temperature anomaly fluctuations also show concordantly but in damped form in the smoothed moving average curves because the curves are historical moving averages with the heaviest weighting toward the more recent years. The lagging responses are also seen at several times when the running average curves variously show warming and cooling for the same year depending on the value of τGLOF .
We posit that the historically filtered warming rate (more than the temperature anomaly) drives GLOF frequency. In Fig. 1 we show GLOF frequency (smoothed over 10-year moving averages) together with the warming rate extracted from the historically filtered temperature and model temperature time series. To get a better match with the temperature treated as such, we applied a further 45-year shift. From a glacier and lake dynamics perspective, this shift might relate to the trigger timescale, τt. Singular values ofτGLOF andτt should not pertain globally to all glaciers but should span wide ranges. The adopted values τGLOF= 80 years and τt= 45 years nonetheless make for a plausible match between the GLOF and climate records. These numbers make sense in terms of glacier and lake dynamics timescales, but we reiterate that our purpose with this climate–GLOF fitting exercise is illustrative. In sum, a notable shift in GLOF frequency does not connote a concordant shift in climate, though prior climate change may still underlie the cause.
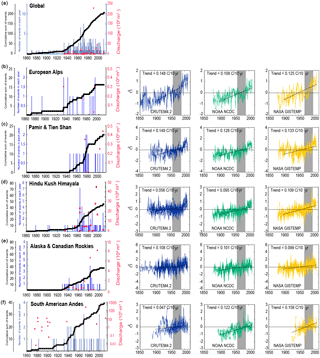
Figure 2(a–f) Left: temporal distribution of regional GLOF frequency and magnitude. At all locations, the cumulative sum of events (black line) indicates an upsurge in the number of events per year. The timing of this upsurge differs by location and likely reflects an increase in reporting, especially in the early part of the record, rather than a change in GLOFs, at least until the 1970s–1990s after which the GLOF rate reduces. Right: global time series climate data from the five regions using CRUTEM 4.2, NOAA NCDC and NASA GISTEMP. Grey columns represent the baseline against which temperature is measured.
Our global analysis identifies 165 moraine-dam GLOFs, recorded since the beginning of the 19th century (Fig. 2a). The vast majority of these GLOFs (n=160; 97 %) occurred since the beginning of the 20th century, at a time of climate warming and increasing glacier recession (Figs. 2 and 5). None of these GLOFs were associated with repeat events from the same lake. Around 65 % of GLOFs occurred between 1930 and 1990. Thirty-six GLOFs occurred in the mountains of western North America between 1929 and 2002 (Table S1 in the Supplement). Fifteen of these occurred in western Canada, 15 in the Cascade Range of the USA and four in Alaska. One occurred in Mexico and 1 in the Sierra Nevada. In the South American Andes we identified 40 GLOFs. Eleven occurred in Chile between 1913 and 2009 (including the large one in Patagonia at Laguna del Cerro Largo in 1989); one in Colombia in 1995 and 28 in Peru between 1702 and 1998. Fourteen GLOFs are listed from the European Alps. Three are from Austria between 1890 and 1940, five from Switzerland between 1958 and 1993 one from France in 1944 and five from Italy between 1870 and 1993. In the Pamir and Tien Shan mountains in central Asia, we identified 20 GLOFs, with most of these dating from the late 1960s to the early 1980s. The largest number of GLOFs (55) is reported from the Hindu Kush Himalaya (HKH) including the mountains of Bhutan and Tibet, dated from the 20th and 21st century. Thirty are from Tibet (between 1902 and 2009), 12 from Nepal between 1964 and 2011(and one is reported to have occurred in 1543) and five in Pakistan between 1878 and 1974. There is uncertainty in reporting some of these GLOFs and we discuss this further in the Supplement.
From around 1930 to about 1950, GLOFs occurred with regularity but a low frequency (Fig. 3). In other words, floods occurred with relatively long period variability (50–60 years). Starting around 1960, the frequency of these events increased (period decreased to approximately 20 years), remaining relatively high until about 1975, after which the statistically significant periodicities end, though GLOFs continue to occur.
While incomplete data restrict a full analysis of GLOF triggers, precise date, magnitude and initiation at a global scale, many GLOFS triggered by ice avalanches and rockfalls occur during summer (see Fig. 4). The characteristics of GLOFs that could be influenced by climate change include changes in magnitude, frequency, timing (either changes in seasonality or changes over longer timescales) and trigger mechanisms. In addition, many rock avalanches into lakes triggering a GLOF may represent a paraglacial response to deglaciation from the LIA or earlier times (Knight and Harrison, 2013; Schaub et al., 2013) and this delayed response demonstrates the need to account for lags between changes in forcing and responses in attribution studies.
From this analysis, we highlight three key observations: (1) GLOFs became more common around 1930 but then their incidence was maintained at a quasi-steady level for a few decades thereafter; (2) since about 1975, GLOF periodicity has decreased globally; and (3) the periodicities of GLOF occurrence have changed throughout the 20th century. These observations are discussed below.
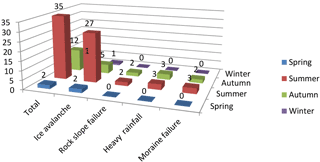
Figure 4Seasonal variation in the occurrence of GLOF associated with the failure of moraine dams. Only a proportion of the GLOFs have seasonal data on timings.
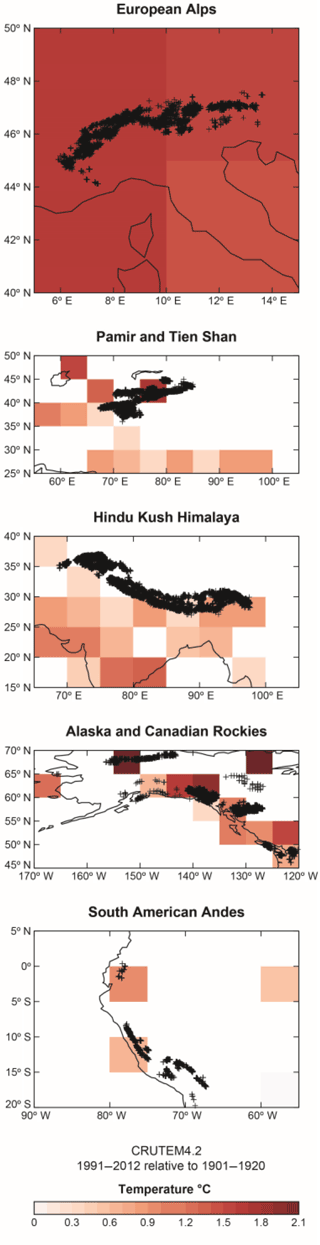
Figure 5Temperature anomalies in the CRUTEM4.2 data set for each mountain region. For each region we extract all the grid points that contain a glacier as defined in the World Glacier Inventory – Extended Format (WGI-XF) and these are shown as black crosses.
Our first main observation is that GLOF frequency increased dramatically and significantly around 1930 globally and between 1930 and 1960 regionally (Figs. 1 and 2). We find no obvious reason for an abrupt improvement of GLOF reporting in 1930. While acknowledging that incompleteness of the record must be a pervasive factor throughout the early period covered by the database, we discount reporting variations as the cause of the abrupt shift. For instance, this pattern is observed in the European Alps, a region with a long history of mountaineering, glacier research and valley-floor habitation and infrastructure development. Given that we record individual GLOFs in the 19th and early 20th centuries we argue that the increase in GLOF frequency in the 1930s represents a real increase rather than an observational artefact. Following the increase around 1930, we observe a similar rate of GLOFs for the subsequent years, typically 1 per year in the following decade, increasing to 2–3 per year during the 1940s (e.g. Figs. 1a, 2a). Again, there is no evidence that incompleteness of data is a main cause of the observed pattern. We therefore conclude that the incidence of global GLOFs has remained generally constant between about 1940 and about 1960. In the 1960s and early 1970s, several years saw more than five GLOFs. We argue below that the trend between 1940 and 1960 hides a more complex spatial and temporal pattern (Clague and Evans, 2000; Schneider et al., 2014).
Our second main observation is that, while there is considerable variability between regions, GLOF incidence rates have decreased since about 1975 globally (Fig. 2). There are both more and larger GLOFs during the 1970s and early 1980s in the Pamir and Tien Shan, in the 1960s in the HKH and in the 1990s in Alaska, the Coast Mountains and Canadian Rockies; and then decreases in both magnitude and frequency follow these periods. In the Andes however, GLOF incidence decreased after the early 1950s. The latter observation may be at least partly attributable to considerable GLOF mitigation measures in Peru, such as engineering-based lake drainage or dam stabilization (Carey et al., 2012; Portocarreo-Rodriguez, 2014). Carrivick and Tweed (2014) propose several reasons why “glacial floods” may have decreased in frequency in recent decades. These include successful efforts to stabilize moraine dams and changes in the ability of fluvial systems to transmit floods over time. We argue, conversely, that this reduction may represent a “lagged” response to glacier perturbations following climate change. More research is clearly needed on this question, and we believe that our analysis, along with that of Carrivick and Tweed's, will stimulate further work and discussion.
Our third main observation is that for several decades in the 20th century, GLOF occurrence has been periodic, but that periodicity has varied. Since about 1975, and especially since 1990, the periodic nature of GLOF occurrence has diminished, even though GLOFs have continued. In other words, GLOFs since 1975 have become more irregular. We suspect that the switch to less periodic outburst floods in recent decades is related to an underlying mechanism such as topographic constraints and glacier hypsometries, with glaciers retreating into steeper slopes, implying a reduced rate of moraine-dammed lake formation – a phenomenon observed, for example, in the European Alps (Emmer et al., 2015).
The statistics of small numbers affect these regional, time-resolved records, but the overall validity of a similar mid-20th century increase and then decrease in the frequency of GLOFs can be further detected in the global record and is statistically significant (Fig. 3). We argue that the reduction in global GLOF frequency after the 1970s (especially in central Asia, HKH and North America) is real, because the contemporary reporting is likely to be nearly complete given the scientific and policy interest in glacier hazards from the late 20th century. Hence, our conclusion is that globally and regionally there have been interdecadal variations in the frequency of GLOFs, and in general the most recent couple of decades have seen fewer GLOFs than the early 1950s to early 1990s. The record's (in)completeness is not able to explain a decreasing incidence rate. This temporal variation in GLOF frequency and recent decrease is therefore a robust and surprising result and has occurred despite the clear trend of continued glacier recession and glacier lake development in recent decades.
Our data allow us to test and refine the widespread assumption that GLOFs are a consequence of recent climate change (Bajracharya and Mool, 2009; Riaz et al., 2014). This is an important assumption because it implies that GLOF frequency will increase as the global climate continues to warm with potential major impacts for downstream regions.
The global increase in GLOF frequency after 1930 must be a response to a global forcing, considering global glacier retreat (Zemp et al., 2015), and physical process understanding suggests that this is a lagged response to the warming marking the end of the LIA (Clague and Evans, 2000). Although the global response appears sudden, in 1930 the region-by-region assessment shows that the response was asynchronous regionally and temporally over three decades (Fig. 2). This is consistent with the fact that the end of the LIA was not globally synchronous (Mann et al., 2009) and we also argue that this reflects regional variations in glacier response times.
We argue that as a climate shift occurs, after some period related to the glacier response time, previously stable or advancing glaciers start to thin and recede; after a further limnological response time proglacial ponds start to grow, coalesce and deepen into substantial moraine-dammed lakes. GLOFs typically occur after some additional period of time (the GLOF response timescale), but this time can be brief in glaciers with short response times, such as in the tropical Andes (Fig. 1).
In the HKH and central Asia the near-concordant formation of many Himalayan glacier lakes and the abrupt increase in GLOF rates in the 1950s and 1960s suggest that the GLOF response time is much smaller than the limnological response time. The moraine evidence here indicates that a shift from mainly glacier advance to recession and/or thinning occurred widely, though regionally asynchronously, between 1860 and 1910. The HKH underwent this shift by around 1860 (Owen, 2009; Solomina et al., 2015) in response to warming following the regional LIA. The limnological response time in the Himalayan–Karakoram region is thus around 100 years, i.e. substantially longer than in the tropical Andes.
We have arrived at a plausible explanation for the post-1930 (1930–1960) increases in GLOF rates. They are most likely heterogeneous, lagging responses to the termination of the LIA, with limnological response times of the order of decades to 100 years, depending on the region (e.g. Emmer et al., 2015). The limnological response times may be of a similar order to the glacier dynamical response times (Jóhannesson et al., 1989; Raper and Braithwaite, 2009) but are appended to them. Thus, measured from a climatic shift to increased GLOFs, the combined glaciological and limnological response times (plus GLOF response times, which may be the shortest of the three response times) may sum to roughly 45–200 years (Fig. 1). It cannot be much more than this, because then we would not see the multi-decadal oscillations in GLOF rates in some regions or globally.
Some individual glaciers may have faster response times than estimated above (Roe et al., 2017), but taken on a broader statistical basis we infer that the most recent GLOFs are a delayed response to the end of the LIA. A fundamental implication is that anthropogenic climatic warming to date will likely manifest in increasing GLOFs in some regions of the world starting early this century and continuing into the 22nd century. In all the mountain regions considered here the available evidence indicates a warming trend over the last century around 0.1 ∘C decade−1 (Figs. 2 and 5). The trend varies between data set and region, with the highest rates in the Pamir Tien Shan region and the lowest in the HKH. The most uncertain region is the Andes, where the sparseness of data prevents any meaningful assessment. The trends are consistent with the global mean land temperature trend 0.95 ± 0.02 to 0.11 ± 0.02 ∘C for 1901–2012, implying these regions have warmed at approximately the same rate as the global land surface.
The baseline behaviour of glacial lake systems in the absence of climate change is not known in detail, but the low rate of GLOFs prior to 1930 may indicate that without warming the frequency would be low. The difficulty of attributing individual GLOF behaviour to climate change relates to the presence of non-climatic factors affecting GLOF behaviour, such as moraine dam geometry and sedimentology, climate-independent GLOF triggers (e.g. earthquakes) and the timescales related to destabilization of mountain slopes, producing mass movements into lakes (Haeberli et al., 2017). This represents the period of paraglaciation (e.g. Ballantyne, 2002; Holm et al., 2004; Knight and Harrison, 2013). These system characteristics may vary regionally and temporally within the evolutionary stage of a receding mountain glacier, and non-climatic factors such as lake mitigation measures additionally influence GLOF frequency and magnitude (Clague and Evans, 2000; Portocarreo-Rodriguez, 2014). We argue that while the original driver of lake development is likely to involve climate change (resulting in glacier downwasting and slowed meltwater flux through glaciers systems as glacier surfaces reduce in gradient) other mechanical and thermodynamic processes likely assume more importance as the lakes evolve, and these includes small-scale calving and insolation-induced melting of ice cliffs (e.g. Watson et al., 2017).
We also recognize that contemporary mountain glaciers are dissimilar to those that existed in the LIA. They are, in the main, shorter, thinner and with prominent moraines. Assumptions that climate processes acted on similar glacial systems over time are therefore likely to be simplistic.
Based on the analysis of our global GLOF database we have shown that a clear trend is detectable globally and regionally diversified in the 20th century with a sharp increase in GLOF occurrence around 1930. This trend is attributable to the observed climate trend, namely the warming since the end of the LIA. The delayed response of GLOF occurrence is an exemplar for the complexities of how natural systems respond to climate change, underlining the challenges of attribution of climate change impacts. We have shown here that attribution of GLOFs to climate change is possible, although the suite of factors influencing GLOF occurrence cannot be fully quantified.
In addition, lake outbursts following moraine failures are likely to be quite different in different regions. This reflects differences in a number of factors including ground thermal conditions, presence or absence of ground ice and permafrost, influence of extreme weather and seismic processes, topography and glacial history. To assess these we would need to better understand the geomorphological timescales involved in lake evolution and failure to design a more robust statistical analysis and to understand each region's GLOF history. We thus recommend close attention by the Earth surface processes science community to various process timescales using field studies, satellite remote sensing and theoretical modelling.
Our inventory and the global pattern of GLOFs that is derived from it lacks in many cases precise data on the processes responsible for GLOFs. This is a consequence of incomplete reporting of GLOFs in remote mountain regions, especially before the advent and wide use of remote sensing. In many cases the record is of a large flood being observed and then some time afterwards a collapsed moraine dam is seen and the flood is attributed to this collapse. Clearly the precise details of how the collapse occurred is not always available, and this uncertainty bedevils all similar detection and attribution studies, especially on those events associated with rapid geomorphological change. This intrinsic incompleteness in the record is problematic but should not prevent reasonable assertions on GLOF triggers to be made, especially if global-scale and consistent patterns in GLOF behaviour are observed.
Future research should therefore more systematically study the factors influencing GLOF frequency and magnitude and lake formation where a distinction between GLOF conditioning and triggering factors will be helpful (e.g. Gardelle et al., 2011).
If climate (such as temperature time series) influences GLOFs, as surely must be the case, long lag times are necessarily implied by the empirical data sets. With such lags as we have modelled, this brings the increase of GLOFs following 1930 into line with temperature increases at the end of the Little Ice Age. Subsequent changes in the GLOF rate (including a several-decades-long fall in GLOF rates) can similarly be attributed to fluctuations in global warming. If these conclusions are broadly correct, a further implication is that an acceleration in GLOF rates will probably occur in the 21st century, perhaps starting rather soon. Even though the actual global warming rate for the 21st century may be nearly constant, as modelled, the fitted warming rate as plotted in Fig. 1f accelerates because of the memory of a post-LIA, pre-anthropogenic quasi-stable climate. We are entering a stage where anthropogenic warming will increasingly dominate GLOF activity and attribution of GLOFs to anthropogenic global warming will be confirmed. For now, this remains a hypothetical projection or expectation and is not yet borne out in the GLOF record.
We conclude that the global record of GLOF following the failure of moraine dams shows a dramatic increase in GLOF occurrences from 1930 to 1970, then a decline. We also observe that the GLOF frequency has not fluctuated directly in response to global climate. A reasonable premise is that climate, glaciers, glacier lakes and GLOFs are closely connected, but the connections between climate and GLOFs are hidden in response time dynamics. We argue that response times do not necessarily reflect linear processes and that lake growth may result in none, single or multiple GLOFs from the same lake systems. Accordingly, the response times must vary widely from region to region and glacier to glacier. From this we infer that the 1930 to 1970 increase in global GLOF activity is likely a delayed response following warming that ended the LIA and decreased the rate of moraine-dammed lake formation. We also infer that the decrease in GLOF frequency after 1970 is likely related to a delayed response to the stabilization of climate following the LIA. In addition, a minor cause (though important locally, for instance in Peru and Switzerland in particular), GLOF mitigation engineering, may have circumvented a few GLOFs, thus contributing to the downward trend in recent decades. We can expect a substantial increase in GLOF incidence throughout the 21st century as glaciers and lakes respond more dynamically to anthropogenic climate warming. This is corroborated by recent modelling studies projecting the location, number and dimension of new lakes in areas where glacier will recede over the coming decades in the Alps, the Himalayas or the Andes (Linsbauer et al., 2016).
As a result, we argue that the sharply increased GLOF rates starting from 1930 followed by reduced GLOF frequency from high levels in the mid-20th century are both real and we speculate these trends may reflect the failure of sensitive glacial lake systems in a lagged response to initial glacier recession from LIA limits. The apparent robustness of contemporary lake systems suggests that only the most resilient moraine-dammed lakes have survived recent climate change. Predicting their future behaviour is of great importance for those living and working in mountain communities and those developing and planning infrastructure in such regions.
The database of GLOFs analysed in this paper is attached as a supplement.
The supplement related to this article is available online at: https://doi.org/10.5194/tc-12-1195-2018-supplement.
The project was designed by SH following discussions with JK, CH and JR. Climate model data were provided by AW and RAB. Data analysis was carried out by SH, JK, DHS, LR and UH. JR, VV and AE provided inventory data. All authors helped to write and review the text.
The authors declare that they have no conflict of interest.
Stephan Harrison was funded by a Leverhulme Research Fellowship.
Stephan Harrison, Richard A. Betts and Andy Wiltshire acknowledge funding
under the HELIX (European Union Seventh Framework Programme FP7/2007-2013
under grant agreement no. 603864). Andy Wiltshire and Richard A. Betts
acknowledge funding from the Joint UK DECC/Defra Met Office Hadley Centre
Climate Programme (GA01101). John Kennedy of the Met Office Hadley Centre
provided advice on handling the temperature observation data sets used in this
project. Contributions by Jeffrey S. Kargel, Umesh K. Haritashya,
Dan H. Shugar, and Dhananjay Regmi were supported by NASA's Understanding
Changes in High Mountain Asia programme, the NASA/USAID SERVIR Applied Science
Team programme, and by the United Nations Development Program. We thank
C. Scott Watson and an anonymous reviewer for their detailed and incisive
reviews of the paper. We also thank Georg Veh, Jonathan Carrivik and Sergey
Chernomorets for further comments and clarifications of the
inventory.
Edited by: Chris R.
Stokes
Reviewed by: C. Scott Watson and one anonymous referee
Bajracharya, S. R. and Mool, P.: Glaciers, glacial lakes and glacial lake outburst floods in the Mount Everest region, Nepal, Ann. Glaciol., 50, 81–86, 2009.
Bahr, D. B., Pfeffer, W. T., Sassolas, C., and Meier, M. F.: Response time of glaciers as a function of size and mass balance, J. Geophys. Res., 103, 9777–9782, 1998.
Ballantyne, C. K.: Paraglacial geomorphology, Quaternary Sci. Rev., 21, 1935–2017, 2002.
Carey, M.: Living and dying with glaciers: people's historical vulnerability to avalanches and outburst floods in Peru, Global Planet. Change, 47, 122–134, 2005.
Carey, M., Huggel, C., Bury, J., Portocarrero, C., and Haeberli, W.: An integrated socio-environmental framework for glacier hazard management and climate change adaptation: lessons from Lake 513, Cordillera Blanca, Peru, Clim. Change, 112, 733–767, 2012.
Carrivick, J. L. and Tweed, F. S.: A global assessment of the societal impacts of glacier outburst floods, Global Planet. Change, 144, 1–16, 2016.
Carrivick, J. L., Russell, A., and Tweed, F. S.: Geomorphological evidence for jökulhlaups from Kverkfjöll volcano, Iceland, Geomorphology, 63, 81–102, 2004.
Clague, J. J., Evans, S. G., and Blown, I. G.: A debris flow triggered by the breaching of a moraine-dammed lake, Klattasine Creek, British Columbia, Can. J. Earth Sci., 22, 1492–1502, 1985.
Clague, J. J. and Evans, S. G.: A review of catastrophic drainage of moraine-dammed lakes in British Columbia, Quaternary Sci. Rev., 19, 1763–1783, 2000.
Costa, J. E. and Schuster, R. L.: The formation and failure of natural dams, Geol. Soc. Am. Bull., 100, 1054–1068, 1988.
Cramer, W., Yohe, G., Auffhammer, M., Huggel, C., Molau, U., Assuncao Fuas da Silva Dias, M., Solow, A., Stone, D., and Tibig, L.: Detection and attribution of observed impacts, in: Climate Change 2014: Impacts, Adaptation, and Vulnerability, Part A: Global and Sectoral Aspects, Contribution of Working Group II to the Fifth Assessment Report of the Intergovernmental Panel on Climate Change, edited by: Field, C. B., Barros, V., Dokken, D. J., Mastrandrea, M. D., Mach, K. J., Cambridge University Press, Cambridge, UK, and New York, NY, USA, 2014.
Ding, Y. and Liu, J.: Glacier lake outburst flood disasters, China Ann. Glaciol., 16, 180–184, 1992.
Dunning, S. A., Large, A. R. G., Russell, A. J., Roberts, M. J., Duller, R., Woodward, J., Mériaux, A.-S., Tweed, F. S., and Lim, M.: The role of multiple glacier outburst floods in proglacial landscape evolution: The 2010 Eyjafjallajökull eruption, Iceland, Geology, 41, 1123–1126, 2013.
Emmer, A.: Geomorphologically effective floods from moraine-dammed lakes in the Cordillera Blanca, Peru, Quaternary Sci. Rev., 177, 220–234, 2017.
Emmer, A., Merkl, S., and Mergili, M.: Spatio-temporal patterns of high-mountain lakes and related hazards in western Austria, Geomorphology, 246, 602–616, 2015.
Emmer, A., Vilímek, V., Huggel, C., Klimeš, J., and Schaub, Y.: Limits and challenges to compiling and developing a database of glacial lake outburst floods, Landslides, 13, 1579–1584, 2016.
Evans, S. F.: The breaching of moraine-dammed lakes in the southern Canadian Cordillera, Proceedings of the International Symposium on Engineering Geological Environment in Mountainous Areas, Beijing, China, 4–8 May 1987, 2, 141–159, 1987.
Fujita, K., Sakai, A., Nuimura, T., Yamaguchi, S., and Sharma, R. R.: Recent changes in Imja glacial lake and its damming moraine in the Nepal Himalaya revealed by in situ surveys and multi-temporal ASTER imagery., Environ. Res. Lett., 4 045205, https://doi.org/10.1088/1748-9326/4/4/045205, 2012.
Gardelle, J., Arnaud, Y., and Berthier, E.: Contrasted evolution of glacial lakes along the Hindu Kush Himalaya mountain range between 1990 and 2009, Global Planet. Change, 75, 47–55, 2011.
Haeberli, W., Schaub, Y., and Huggel, C.: Increasing risks related to landslides from degrading permafrost into new lakes in de-glaciating mountain ranges, Geomorphology, 293, 405–417, 2017.
Hansen, J., Ruedy, R., Sato, M., and Lo, K.: Global surface temperature change, Rev. Geophys., 48, RG4004, https://doi.org/10.1029/2010RG000345, 2010.
Harrison, S., Glasser, N., Winchester, V., Haresign, E., Warren, C., and Jansson, K.: A glacial lake outburst flood associated with recent mountain glacier retreat, Patagonian Andes, The Holocene, 16, 611–620, 2006.
Holm, K., Bovis, M., and Jakob, M.: The landslide response of alpine basins to post-Little Ice Age glacial thinning and retreat in southwestern British Columbia, Geomorphology, 57, 201–216, 2004.
Huggel, C., Kääb, A., Haeberli, W., Teysseire, P., and Paul, F.: Remote sensing based assessment of hazards from glacier lake outbursts: a case study in the Alps, Can. Geotech. J. 39, 316–330, 2002.
Iribarren, P. A., Mackintosh, A., and Norton, K. P.: Hazardous processes and events from glacier and permafrost areas: lessons from the Chilean and Argentinean Andes, Earth Surf. Proc. Land., 40, 2–21, https://doi.org/10.1002/esp.3524, 2014.
Ives, J. D., Shrestha, R. B., and Mool, P. K.: Formation of glacial lakes in the Hindu Kush-Himalayas and GLOF risk assessment, International Centre for Integrated Mountain Development (ICIMD), Kathmand, available at: http://www.indiaenvironmentportal.org.in/files/ICIMODGLOF.pdf, 2010.
Jiang, Z. X., Cui, P., and Jiang, L. W.: Critical hydrologic conditions for overflow burst of moraine lake, Chinese Geogr. Sci., 14, 39–47, 2004.
Jóhannesson, T., Raymond, C., and Waddington, E.: Time scale for adjustment of glaciers to changes in mass balance, J. Glaciol., 35, 355–369, 1989.
Jones, P. D., Lister, D. H., Osborn, T. J., Harpham, C., Salmon, M., and Morice, C. P.: Hemispheric and large-scale land surface air temperature variations: An extensive revision and an update to 2010, J. Geophys. Res., 117, D05127, https://doi.org/10.1029/2011JD017139, 2012.
Kargel, J. S., Leonard, G. J., Bishop, M. P., Kaab, A., and Raup B. (Eds): Global Land Ice Measurements from Space (Springer-Praxis), Heidelberg, https://doi.org/10.1007/978-3-540-79818-7, 2014.
Kershaw, J. A., Clague, J. J., and Evans, S. G.: Geomorphic and sedimentological signature of a two-phase outburst flood from moraine-dammed Queen Bess lake, British Columbia, Canada, Earth Surf. Proc. Land., 30, 1–25, 2005.
Knight, J. and Harrison, S.: The impacts of climate change on terrestrial Earth surface systems, Nat. Clim. Change, 3, 24–29, 2013.
Korup, O. and Tweed, F.: Ice, moraine, and landslide dams in mountainous terrain, Quaternary Sci. Rev., 26, 3406–3422, 2007.
Lamsal, D., Sawagaki, T., Watanabe, T., and Byers, A. C.: Assessment of glacial lake development and prospects of outburst susceptibility: Chamlang South Glacier, eastern Nepal Himalaya, Geomat. Nat. Haz. Risk, 7, 403–423, https://doi.org/10.1080/19475705.2014.931306, 2014.
Lane, S. N.: Assessment of rainfall-runoff models based upon wavelet analysis, Hydrol. Process., 21, 586–607, 2007.
Linsbauer, A., Frey, H., Haeberli, W., Machguth, H., Azam, M. F., and Allen, S.: Modelling glacier-bed overdeepenings and possible future lakes for the glaciers in the Himalaya – Karakoram region, Ann. Glaciol., 57, 119–130, 2016.
Lliboutry, L., Arnao, B. M., and Schneider, B.: Glaciological problems set by the control of dangerous lakes in Cordillera Blanca, Peru III: Study of moraines and mass balances at Safuna, J. Glaciol., 18, 275–290, 1977.
Mann, M. E., Zhang, Z., Hughes, M. K., Bradley, R. S., Miller, S. K., and Rutherford, S.: Proxy-Based Reconstructions of Hemispheric and Global Surface Temperature Variations over the Past Two Millennia, P. Natl. Acad. Sci. USA, 105, 13252–13257, 2008.
Mann, M. E., Zhang, Z., Rutherford, S., Bradley, R., Hughes, M. K., Shindell, D., Ammann, C., Faluvegi, G., and Ni, F.: Global signatures and dynamical origins of the Little Ice Age and Medieval Climate Anomaly, Science, 326, 1256–1260, 2009.
Marzeion, B., Cogley, J. G., Richter, K., and Parkes, D.: Attribution of global glacier mass loss to anthropogenic and natural causes, Science, 345, 919–921, https://doi.org/10.1126/science.1254702, 2014.
Mergili, M. and Schneider, J. F.: Regional-scale analysis of lake outburst hazards in the southwestern Pamir, Tajikistan, based on remote sensing and GIS, Nat. Hazards Earth Syst. Sci., 11, 1447–1462, https://doi.org/10.5194/nhess-11-1447-2011, 2011.
Mool, P. K., Maskey, P. R., Koirala, Joshi, S. P., Lizong, W., Shrestha, A. B., Eriksson, M., Gurung, B., Pokharel, B., Khanal, N. R., Panthi, S., Adhikari, T., Kayastha, R. B., Ghimire, P., Thapa, R., Shrestha, B., Shrestha, S., and Shrestha, R. B.: Glacial Lakes and Glacial Lake Outburst Floods in Nepal, International Centre for Integrated Mountain Development, Kathmandu, 99 pp., available at: http://www.icimod.org/dvds/201104_GLOF/reports/final_report.pdf, 2011.
Narama, C., Severskiy, I., and Yegorov, A.: Current state of glacier changes, glacial lakes, and outburst floods in the Ile Ala-Tau and Kungoy Ala-Too ranges, northern Tien Shan Mountains, Ann. Hokkaido Geogr., 84, 22–32, https://doi.org/10.7886/hgs.84.22, 2010.
O'Connor, J. E., Hardison, J. H., and Costa, J. E.: Debris flows from failures of Neoglacial-age moraines in the Three Sisters and Mount Jefferson wilderness areas, Oregon, US 1803 Geological Survey Professional Paper 1606, 2001.
Oerlemans, J.: Extracting a Climate Signal from 169 Glacier Records, Science, 308, 675–677, https://doi.org/10.1126/science.1107046, 2005.
Osti, R. and Egashira, S.: Hydrodynamic characteristics of the Tam Pokhari Glacial Lake outburst flood in the Mt. Everest region, Nepal, Hydrol. Proc., 23, 2943–2955, 2009.
Owen, L. A.: Latest Pleistocene and Holocene glacier fluctuations in the Himalaya and Tibet, Quaternary Sci. Rev., 28, 2150–2164, 2009.
Perov, V., Chernomorets, S., Budarina, O., Svernyuk, E., and Leontyeva, T.: Debris flow hazards for mountain regions of Russia: regional features and key events, Nat. Hazards, 88, S199–S235, 2017.
Portocarreo-Rodriguez, C. A. and the Engility Corporation: The Glacial Lake Handbook: Reducing risk from dangerous glacial lakes in the Cordillera Blanca, Peru, 68 pp., 2014.
Raper, S. C. B. and Braithwaite, R. J.: Glacier volume response time and its links to climate and topography based on a conceptual model of glacier hypsometry, The Cryosphere, 3, 183–194, https://doi.org/10.5194/tc-3-183-2009, 2009.
Raymond, M., Wegmann, M., and Funk, M.: Inventar gefährlicher Gletscher in der Schweiz, Mitt. VAW/ETH, reprot no. 182, 368 pp., 2003.
Reynolds, J. M.: The identification and mitigation of glacier-related hazards: examples from the Cordillera Blanca, Peru, in: McCall, G. J. H., Laming, D. C. J., and Scott, S., Geo-hazards, London, Chapman & Hall, 143–157, 1992.
Reynolds, J. M. and Richardson, S.: Geological Hazards – Glacial Natural Disaster Management, A presentation to commemorate the International Decade for Natural Disaster Reduction (IDNDR) 1990–2000, 2000.
RGSL: Ongoing efforts to detect, monitor and mitigate the effects of GLOFs in Nepal, Reynolds Geo-Sciences Ltd, Project No. J9622.021, 1997.
RGSL: Glacial hazard assessment for the Upper Indus Basin, Pakistan, Reynolds Geo-Sciences Ltd, J02134, 2002.
Riaz, S., Ali, A., and Baig, M. N.: Increasing risk of glacial lake outburst floods as a consequence of climate change in the Himalayan region, Jàmbá, J. Disaster Risk Studies, 6, 110, https://doi.org/10.4102/jamba.v6i1.110, 2014.
Richardson, S. D. and Reynolds, J. M.: An overview of glacial hazards in the Himalayas, Quaternary Int., 65/66, 31–47, 2000.
Roberts, M., Tweed, F., Russell, A., Knudsen, O., and Harris, T.: Hydrologic and geomorphic effects of temporary ice-dammed lake formation during Jökulhlaups, Earth Surf. Proc. Land., 28, 723–737, 2003.
Roe, G. H, Baker, M. B., and Herla, F.: Centennial glacier retreat as categorical evidence of regional climate change, Nature Geosci., 10, 95–99, 2017.
Russell, A., Tweed, F. S., Roberts, M. J, Harris, T. D., Gudmundsson, M. T., Knudsen, O., and Marren, P. M.: An unusual jökulhlaup resulting from subglacial volcanism, Sólheimajökull, Iceland, Quaternary Sci. Rev., 29, 1363–1381, 2010.
Schaub, Y., Haeberli, W., Huggel, C., Künzler, M., and Bründl, M.: Landslides and new lakes in deglaciating areas: a risk management framework, in: Landslide Science and Practice, edited by: Margottini, C., Canuti, P., and Sassa, K., Springer Berlin Heidelberg, 31–38, https://doi.org/10.1007/978-3-642-31313-4_5, 2013.
Schneider, D., Huggel, C., Cochachin, A., Guillén, S., and García, J.: Mapping hazards from glacier lake outburst floods based on modelling of process cascades at Lake 513, Carhuaz, Peru, Adv. Geosci., 35, 145–155, 2014.
Shugar, D. H. and Clague, J. J.: The sedimentology and geomorphology of rock avalanche deposits on glaciers, Sedimentology, 58, 1762–1783, 2011.
Shugar, D. H., Kostaschuk, R., Best, J. L., Parsons, D. R., Lane, S. N., Orefeo, O., and Hardy, R. J.: On the relationship between flow and suspended sediment transport over the crest of a sand dune, Río Paraná, Argentina, Sedimentology, 57, 252–272, 2010.
Shugar, D. H., Clague, J. J., Best, J. L., Schoof, C., Willis, M. J., Copland, L., and Roe, G. H.: River piracy and drainage basin reorganization led by climate-driven glacier retreat, Nat. Geosci., 10, 370–375, https://doi.org/10.1038/ngeo2932, 2017.
Smith, T. M., Reynolds, R. W., Peterson, T. C., and Lawrimore, J. L.: Improvements to NOAA's Historical Merged Land-Ocean Surface Temperature Analysis (1880–2006), J. Climate, 21, 2283–2293, 2008.
Solomina, O., Bradley, R., Hodgson, D., Ivy-Ochs, S., Jomelli, V., Mackintosh, A., Nesje, A., Owen, L., Wanner, H., Wiles, G., and Young, N.: Holocene glacier fluctuations, Quaternary Sci. Rev., 111, 9–34, 2015.
Stone, D., Auffhammer, M., Carey, M., Hansen, G., Huggel, C., Cramer, W., Lobell, D., Molau, U., Solow, A., Tibig, L., and Yohe, G.: The challenge to detect and attribute effects of climate change on human and natural systems, Clim. Change, 121, 381–395, 2013.
Tweed, F. S. and Russell, A. J.: Controls on the formation and sudden drainage of glacier-impounded lakes: implications for jökulhlaup characteristics, Prog. Phys. Geog., 23, 79–110, 1999.
University of Oslo: Database of glacier and permafrost disasters, University of Oslo, Department of Geosciences, http://www.gaphaz.org/, 2013.
Vilímek, V., Emmer, A., Huggel, Ch., Schaub, Y., and Würmli, S.: Database of glacial lake outburst floods (GLOFs) – IPL Project No. 179, Landslides, 11, 161–165, 2014.
Walder, J. S. and Costa, J. E.: Outburst floods from glacier-dammed lakes: the effect of mode of lake drainage on flood magnitude, Earth Surf. Proc. Land., 21, 701–723, 1996.
Wang, S., Zhang, M., Li, Z., Wang, F., Li, H., Li, Y., and Huand, X.: Glacier area variation and climate change in the Chinese Tianshan Mountains since 1960, J. Geogr. Sci., 21, 263–273, 2011.
Watson, C. S., Quincey, D. J., Carrivick, J. L., and Smith, M. W.: Ice cliff dynamics in the Everest region of the Central Himalaya, Geomorphology, 278, 238–251, 2017.
Westoby, M. J., Glasser, N. F., Hambrey, M. J., Brasington, J., Reynolds, J. M., and Hassan, M.: Reconstructing historic Glacial Lake Outburst Floods through numerical modelling and geomorphological assessment: Extreme events in the Himalaya, Earth Surf. Proc. Land., 39, 1675–1692, 2014.
Wilson, R., Glasser, N. F., Reynolds, J. M., Harrison, S., Anacona, P. I., Schaefer, M., and Shannon, S.: Glacial lakes of the Central and Patagonian Andes, Global Planet. Change, 162, 275–291, https://doi.org/10.1016/j.gloplacha.2018.01.004, 2018.
Worni, R., Stoffel, M., Huggel, C., Volz, C., Casteller, A., and Luckman, B.: Analysis and dynamic modeling of a moraine failure and glacier lake outburst flood at Ventisquero Negro, Patagonian Andes (Argentina), J. Hydrol., 444–445, 134–145, 2012.
Xu, D.: Characteristics of debris flow caused by outburst of glacial lakes on the Boqu River in Xizang, China, J. Glaciol. Geocryol., 9, 23–34, 1987.
Zapata, M. L.: La dinamica glaciar en lagunas de la Cordillera Blanca, Acta Montana, 19, 37–60, 2002.
Zemp, M., Frey, H., Gärtner-Roer, I., Nussbaumer, S. U., Hoelzle, M., Paul, F., Haeberli, W., Denzinger, F., Ahlstrøm, A. P., Anderson, B., and Bajracharya, S.: Historically unprecedented global glacier decline in the early 21st century, J. Glaciol., 61, 745–762, 2015.