the Creative Commons Attribution 4.0 License.
the Creative Commons Attribution 4.0 License.
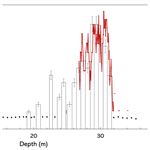
Temporal markers in a temperate ice core: insights from 3H and 137Cs profiles from the Adamello Glacier
Elena Di Stefano
Giovanni Baccolo
Massimiliano Clemenza
Barbara Delmonte
Deborah Fiorini
Roberto Garzonio
Margit Schwikowski
Valter Maggi
The article discusses the use of tritium (3H) and cesium (137Cs) as temporal markers in ice cores extracted from temperate glaciers. We present a complete tritium profile for a 46 m ice core drilled from the Adamello Glacier, a temperate glacier in the Italian Alps, and compare it to the 137Cs profile from the same ice core. Our analysis reveals tritium contamination between 19 and 32 m of depth, which can be attributed to the global radioactive fallout caused by atmospheric nuclear-bomb testing that took place in the 1950s and 1960s. Results show that the radioactive peak associated with 1963 does not occur at the same depth for both 3H and 137Cs; instead, the tritium peak is 1.5 m above the cesium one. Our hypothesis is that this misalignment is caused by meltwater-induced post-depositional processes that affect 137Cs, which is more sensitive to percolation than 3H. The total inventory of 137Cs in this ice core is also among the lowest ever reported, providing additional evidence that the presence of meltwater has affected the distribution of this radionuclide within the ice. In contrast, the total tritium inventory is comparable to what is reported in the literature, making it a more reliable temporal marker for temperate glaciers.
- Article
(1177 KB) - Full-text XML
- BibTeX
- EndNote
Due to its sensitivity to temperature variations, the cryosphere offers some of the most notable evidence of climate change, with dramatic ice losses reported worldwide. In particular, mountain glaciers are losing mass at accelerating rates globally (Zemp et al., 2019), and according to future projections, including the most optimistic ones, this trend will continue throughout the current century (Hock et al., 2019). European Alpine glaciers are among the fastest declining glaciers, with an estimated average annual ice loss of m of water equivalent for the period 2000–2016 (Davaze et al., 2020). As a result of increasing temperatures, glaciers are not only retreating but also undergoing changes in their thermal regimes. Atmospheric warming and the increasing number of melting events are rapidly modifying the temperature distribution within glaciers, increasing ice temperatures (Gilbert et al., 2010, 2020). The occurrence of accumulation basins with temperatures consistently below the pressure melting point is becoming increasingly rare in many mountain ranges as they transition from cold to temperate conditions (Gabrielli et al., 2010). Temperate ice is defined as ice that can contain meltwater inclusions due to its thermodynamic state (Lliboutry, 1971). Moreover, the rise in the equilibrium-line altitude (ELA), defined here as the altitude at which a glacier has a zero mass balance, is leading to a gradual reduction in glacier size, ultimately resulting in the disappearance of accumulation basins. It is expected that by the end of the 21st century, at least 69 % of the glaciers in the Alps will be entirely below the ELA (Zebre et al., 2021). As a consequence of increased temperatures and the subsequent percolation of meltwater, it is becoming increasingly difficult to retrieve reliable climatic records from mountain glaciers through ice-core drilling. In the future, the role of glaciers as paleoclimatic and environmental archives will depend on our ability to interpret signals from temperate glaciers. Determining if and to what degree such glaciers can provide climatic and environmental records is an urgent issue that ice-core science must address. Meltwater percolation poses a potential threat to the preservation of proxy signals in the ice. Possible effects include smoothing the isotopic signal (Thompson et al., 1993), relocating impurities (Pavlova et al., 2015), and altering trace element records. Because meltwater-induced post-depositional effects vary among chemical species and can be influenced by several factors (Avak et al., 2018; Moser et al., 2023), such as solubility, position within the ice lattice, and concentration, it can sometimes be difficult to evaluate to what degree the signal has been altered. Generally, elements and compounds that are insoluble in water, present in particle form, or well incorporated into the ice lattice will be less prone to elution and relocation due to meltwater (Eichler et al., 2001; Wong et al., 2013). Microparticles may still accumulate at melt surfaces, leading to the enrichment of elements bound to particulate matter (Pavlova et al., 2015; Niu et al., 2017).
Among the many contaminants we can find in ice cores, there are radionuclides of anthropogenic origin. During the 1950s and 1960s, atmospheric nuclear-bomb testing, mainly carried out by the USA and USSR, resulted in global radioactive contamination of the environment. Atmospheric thermonuclear explosions can reach the stratosphere, where circulation allows the contamination to rapidly spread across the entire hemisphere. The majority of tests were carried out in the Northern Hemisphere, which accordingly suffers from the highest contamination, although radionuclides generated from atmospheric nuclear tests have been found in the Antarctic continent as well (Picciotto and Wilgain, 1963; Jouzel et al., 1979). In 1963, the atmospheric yield of nuclear-bomb testing reached its maximum (United Nations Scientific Committee on the Effects of Atomic Radiation, 2000), before the Partial Test Ban Treaty, which introduced a ban on atmospheric nuclear testing for the USA, USSR, and UK, came into force. This maximum has been recorded in paleoclimatic archives and has subsequently been used as a temporal reference horizon (Mikhalenko et al., 2015; Eichler et al., 2000; Clemenza et al., 2012). Likewise, radioactive layers corresponding to the 1986 Chernobyl accident (United Nations Scientific Committee on the Effects of Atomic Radiation, 2008; International Atomic Energy Agency, 2006) and the 2011 Fukushima accident (Steinhauser et al., 2014) have been found in environmental records. The main radionuclides injected into the environment following such events include cesium (137Cs) and tritium (3H), both of which have been extensively documented in glaciers and ice caps. The radioactive layers corresponding to 1963 and 1986 can assist in the dating of ice cores if the signals are well preserved and can shed some light on melting processes within the glaciers (Kang et al., 2015).
Here, we present the complete 3H profile for a 46 m ice core extracted from the Adamello Glacier, a low-altitude temperate glacier. To assess the integrity of signals in temperate ice, the tritium record is also compared with 137Cs and hyperspectral data related to dust content. Finally, a comparison is made with similar data from a cold glacier (Colle del Lys).
1.1 Tritium
Tritium is a pure beta emitter with a maximum emission energy of 18 keV and a half-life of 12.33 years (Lucas and Unterweger, 2000). It is naturally produced in the upper atmosphere as a result of the interaction between cosmic rays and atmospheric gases, mainly nitrogen, at a production rate of 0.320 atoms cm−2 s−1 (Masarik and Beer, 2009). This results in a low natural background of tritium that is detectable in precipitation, estimated to be <1 Bq L−1 in the United States (Thatcher, 1962; Kaufman and Libby, 1954), although pre-1950s measurements, taken before the artificial production of tritium began, are quite scarce. Reconstructions of the natural background of tritium are available from ice-core data from the Fiescherhorn glacier (Schotterer et al., 1998a).
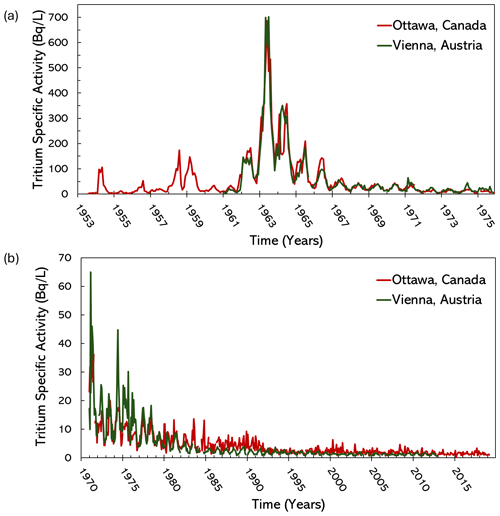
Figure 1Monthly concentrations of tritium in precipitation in Vienna (Austria) and Ottawa (Canada). Panel (a) shows data from 1953 to 1975, while panel (b) shows data from 1971 to 2019. These data were obtained from the Global Network of Isotopes in Precipitation (GNIP) database (International Atomic Energy Agency and World Meteorological Organization, 2021) and converted to Bq L−1 (where 1 tritium unit (TU) = 0.118 Bq L−1). Please note the different scales on the y axes in the two panels.
3H was a key component in thermonuclear bombs, serving as one of the hydrogen isotopes used as thermonuclear fuel. During the nuclear testing period, tritium concentrations in Northern Hemisphere precipitation reached several thousand tritium units (TU). Figure 1 shows monthly concentrations of tritium in precipitation for Vienna and Ottawa, obtained from the Global Network of Isotopes in Precipitation (GNIP) database (International Atomic Energy Agency and World Meteorological Organization, 2021). These trends, representative of the Northern Hemisphere, show a gradual increase during the 1950s that leads up to a maximum in 1963, followed by a gradual decrease after the enactment of the Partial Test Ban Treaty. Tritium activity concentrations show strong seasonality, with peak values occurring in spring–summer each year. This phenomenon, known as the “spring leak” (Michel, 2005; Harms et al., 2016), is due to enhanced air exchange between the troposphere and the stratosphere, the main reservoir for this radionuclide, during spring. 3H has been extensively used as a temporal horizon marker for 1963 in ice cores (Eichler et al., 2000; Qiao et al., 2021; Mikhalenko et al., 2015; Gabrieli et al., 2011; Kang et al., 2015) and, if activities were high, to reveal seasonal signals and aid annual-layer counting in ice cores (Schwikowski et al., 1999; Schotterer et al., 1998b; Yasunari et al., 2007). Given tritium's relatively short half-life, its detection is gradually becoming more challenging as more time passes since its emission into the environment.
1.2 Adamello ice core
An ice core (hereafter referred to as ADA16) was drilled during the spring of 2016 in Pian di Neve (46°8′51′′ N, 10°31′22′′ E), the former accumulation basin for the Adamello Glacier; this glacier has experienced several years of negative mass balance (Ranzi et al., 2010) and is now entirely located below the ELA. The first chronology for this ice core estimated a surface age of 1993, indicating no accumulation has been preserved on this glacier for the last 20 years (Festi et al., 2021). Due to its relatively low altitude (3100 m a.s.l.) and prevailing climatic conditions, the Adamello Glacier has a temperate regime – the body of the glacier is at the melting point, and during summer, it is subject to significant melting, which could alter the climatic signal preserved in the ice. The ice core was drilled at the estimated location of the maximum ice thickness of the glacier, 268±5 m (Picotti et al., 2017), and reached a final depth of 46 m. During drilling, ice-core chips were also collected. These are residual materials produced during mechanical drilling due to the contact between the ice and the rotating blade, and they are usually discarded due to potential chemical contamination resulting from the ice being in direct contact with the drill. Nonetheless, they are suitable for radionuclide analysis as radioactive contamination during drilling operations is virtually impossible, even though they have poor depth resolution, limited to the entire length of the single ice-core sections extracted during drilling runs. The chips were collected in a specific closed chamber above the core barrel, which was emptied after the collection of each core sample.
Analyses were performed both on the chips and on ice samples from the ice core. The chips were divided into 112 runs, with some stored together, for a total of 71 samples, each covering a mean thickness of 59.4 cm. Ice samples were taken from 27 to 35 m of depth to obtain a higher temporal resolution for this part of the ice core, with samples collected at intervals of 5 to 10 cm.
1.3 Lys ice core
To have a reference from a cold Alpine glacier, we also analyzed 38 samples from an ice core drilled at the Colle del Lys site (45°55′13′′ N, 7°51′4′′ E), which constitutes the uppermost part of the Lys Glacier and is located in the Monte Rosa massif at 4240 m above sea level (a.s.l). This is one of the few glacierized areas in the Alps where cold ice can be found. Thanks to their high elevation, glaciers in the upper regions of the Monte Rosa massif are only occasionally subjected to melting (Hoelzle et al., 2011). Consequently, several undisturbed ice cores have been retrieved from this area, specifically from Colle del Lys and Colle Gnifetti (Smiraglia et al., 2000; Villa et al., 2006; Wagenbach et al., 2012). For the purposes of this study, we analyzed remaining samples from the 137Cs analysis carried out by Clemenza et al. (2012).
2.1 Sample preparation and analysis
Samples from the ADA16 ice core were thawed in a clean room inside precleaned polyethylene bottles and then filtered using a vacuum-driven filtration system and polycarbonate filters (diameter: 47 mm; cutoff: 0.45 µm). They were then passed through a tritium column (Eichrom's Tritium Column) to eliminate any possible radioisotopic disturbances present in the sample. The tritium columns were first conditioned using 10 mL of ultrapure water before adding 25 mL of the sample, of which the first 5 mL was discarded, while the rest was collected for further preparation. Finally, samples were prepared for liquid scintillation counting by mixing 8 mL of the sample with 12 mL of a scintillation cocktail (Ultima Gold LLT) in a 20 mL low-diffusion polypropylene vial.
Samples from the Lys Glacier were directly prepared for liquid scintillation and measured using a total-beta protocol. Data from this ice core will be presented in counts per minute (cpm) due to the inability to construct a calibration curve for total-beta analysis.
The liquid scintillation counter used was the Quantulus 1220, manufactured by PerkinElmer. It is equipped with two dual multichannel analyzers, enabling the simultaneous measurement of four spectra, each with a resolution of 1024 channels. In our analysis, we considered a counting window between channels 50 and 250, which covers the emission energy of tritium.
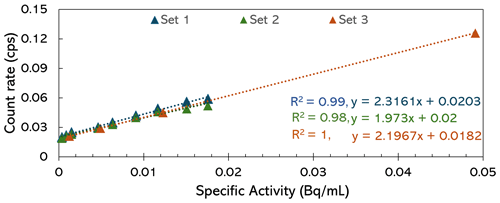
Figure 2Calibration curves calculated for the three sets of tritium standards. Data points are plotted in terms of counts per second (cps) vs. activity values (Bq mL−1). For each set, the dotted line represents the linear fit between the data points, which is also reported in the bottom-right corner of the image along with the coefficient of determination (R2). Errors in the count rate, calculated as the square root of each measurement divided by the count time, amount to 0.001 cps.
2.2 Calibration curves and detection limit
For conversion from the count rate (cps) to the specific activity (Bq L−1), we constructed a calibration curve for each dataset (low-resolution chips and a high-resolution ice core) by measuring tritium standards of a known activity, as can be seen in Fig. 2. Standards were prepared using the National Institute of Standards and Technology (NIST) Hydrogen-3 Radioactivity Standard 4361C. Three sets of standards (Set1, Set2, and Set3) were prepared following the same procedure that was used for the samples, with the only exception being that Set2 was the only one that passed through the tritium columns. Set1 and Set2 were prepared with identical activities to estimate the potential influence of the elution columns on sample activities. As the difference between Set1 and Set2 was below 15 % (in terms of cps), we decided not to elute the tritium standards. Set3 was repeatedly measured between sample runs to check for instrumental stability. Set1 was used for calibration of the low-resolution dataset, while Set3 was employed for the high-resolution dataset. The errors in the count rate were calculated as the square root of each measurement divided by the count time.
Following Currie (1968), we calculated the critical level (LC) as LC =, where Rb is the background rate and t is the measurement time. When the net sample rate is higher than the critical level, we can consider the sample contribution (Rs − Rb) to be distinct from the background, and the specific activity (Bq L−1) was calculated using the calibration coefficient from the standard measurements. All activities were decay corrected to 1 January 2016, and the associated uncertainty was calculated employing the “propagation of error” method. When (Rs − Rb) < LC, an upper limit was calculated as 3 times the counting error in the sample measurement. The detection limit for our measurement was 1.5 Bq L−1, calculated as 3.3σ, where σ is the standard deviation of 10 independent blanks (Currie, 1968).
2.3 Hyperspectral imaging spectroscopy
Hyperspectral imaging spectroscopy is a technique used to characterize surfaces and materials on the basis of their optical properties. In the visible part of the electromagnetic spectrum (from 300 to 750 nm), snow and ice show high reflectance. Certain ice-core characteristics, such as impurity content (e.g., mineral dust, volcanic ash, black carbon, and algae), affect the ice's capability to absorb and reflect electromagnetic radiation in the visible range, enabling the extraction of a continuous record of reflectance and derived parameters along the ice core. Ice-core sections were placed on a fixed ice-core support, illuminated using a stable halogen light source (600 or 1000 W, Quantum Design Europe), and scanned with a Headwall spectrometer (400–1000 nm, Headwall Photonics Hyperspec Visible Near Infrared (VNIR) hyperspectral imager). The hyperspectral imaging scanner was set in motion step by step from the top to the bottom of the sections using a motion speed of 0.9 mm s−1 and an exposure time per frame of 39.8 ms. The high-resolution images were then processed to obtain reflectance curves focusing on a 20-pixel section centered along the central axis of the ice-core sections. The technique, developed in collaboration between the European Cold Laboratory (EuroCold) and the Remote Sensing of Environmental Dynamics Laboratory (LTDA), both located at University of Milan-Bicocca, is described in detail in Garzonio et al. (2018). For this study, we analyzed a portion of the ice core that was about 7 m long (from 26.8 to 33.9 m), corresponding to the area around the main 137Cs peak. Our objective was to investigate whether the presence of impurities could impact the distribution of radionuclides in the ice, given previous research demonstrating a significant association between cesium and particulate matter (Di Stefano et al., 2019). Finally, we calculated the snow darkening index (SDI) as the normalized ratio between the reflectance in the red wavelengths (i.e., from 640 to 670 nm) and green wavelengths (i.e., from 550 to 590 nm), providing information on the concentration of mineral dust in the ice core (Di Mauro et al., 2015).
3.1 3H activity
The tritium profile for the Adamello ice core is presented in Fig. 3. The low-resolution dataset covered the whole depth of the ice core, but only the portion between 19 and 32 m displayed a tritium signal, implying that these layers can be attributed to the period between the 1950s and the 1970s.
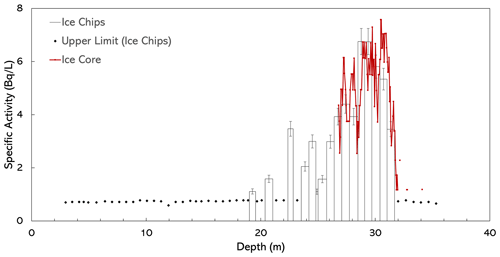
Figure 3Tritium activity in the temperate Adamello ice core. Low-resolution measurements referring to ice chips are shown as grey histograms, where the width of each bar represents the actual depth covered by the sample and the height represents the specific activity (Bq L−1). Error bars indicate the uncertainty in the specific activity, calculated following the propagation of error method. Diamonds represent samples from the low-resolution dataset for which the upper limit was calculated instead of the specific activity, as detailed in the main text. High-resolution measurements referring to ice-core samples are shown in red.
The tritium signal was further investigated by analyzing the high-resolution dataset between 27 and 35 m. In general, good agreement between the two datasets was found regarding both the measured specific activities and the general trend, with the exception of the main peak, which was found at 29±0.6 m in the low-resolution dataset and at 30.530±0.025 m in the high-resolution dataset. We consider the low-resolution dataset to be less reliable due to its broader depth coverage, which may lead to inaccuracies when the signal exhibits peaks and valleys compressed in a narrow depth range, as shown in the high-resolution dataset. Activity values are generally lower than those reported for other Alpine glaciers (Eichler et al., 2000; Schotterer et al., 1998b, 1977; Oerter and Rauert, 1982), even when accounting for decay. Discrepancies between tritium activities in precipitation reported at the time of the radioactive fallout and tritium detected in ice cores can be explained by (i) immediate loss of a fraction of the initial deposited signal due to summer snowmelt, as proposed by Oerter and Rauert (1982), and (ii) current loss due to melting and water percolation within the ice, which is expected to be more extensive in the Adamello ice core than in ice cores extracted from cold glaciers.
3.2 137Cs and 3H profile comparison
It is interesting to compare the tritium signal with the 137Cs profile from the same ice core, as detailed by Di Stefano et al. (2019). 137Cs is also a byproduct of atmospheric nuclear tests, and since the transport mechanisms of these two radionuclides are similar (Pourchet and Pinglot, 1979), it is expected to find the 1963 peak at the same depth for 137Cs and 3H, as observed in ice cores from cold glaciers (Pinglot et al., 2003). We note that, in this study, the low-resolution tritium dataset was prepared from the same exact samples as those used for 137Cs analysis.
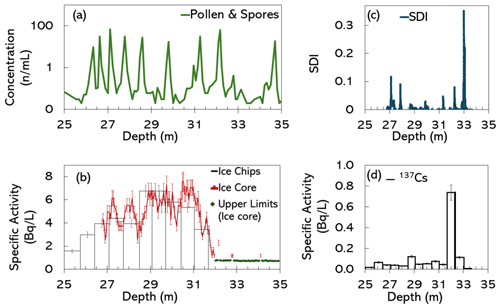
Figure 4Detailed overview of the ice core from the portion where the 1963 peaks of tritium and 137Cs have been observed (between 25 and 35 m of depth). (a) Concentration of pollen and spores taken from Festi et al. (2021). Each peak in the concentration of pollen and spores reflects 1 flowering year (February–September). (b) Tritium profiles. Specific activity (Bq L−1) of 3H for the low-resolution ice-chip dataset is shown in the form of black histograms, while the high-resolution ice-core dataset is shown in red. Error bars indicate the uncertainty in the specific activity, calculated following the propagation of error method. The diamonds indicate upper limits calculated for the high-resolution dataset, as detailed in the main text. (c) Snow darkening index (SDI) record, a hyperspectral index related to the impurity content of ice. (d) Specific activity (Bq L−1) of 137Cs, taken from Di Stefano et al. (2019).
In the Adamello ice core, the respective peaks are misaligned by at least 1.5 m when considering the high-resolution dataset for tritium, as can be seen in Fig. 4b and d. Based on the dating proposed by Festi et al. (2021), this would correspond to a time lag of approximately 2 years between the two peaks, which in terms of deposition would be difficult to explain. Our hypothesis is thus that the 137Cs signal has been altered by post-depositional processes. We must note that Festi et al. (2021) used data from Di Stefano et al. (2019) as a tie point for 1963; therefore, the established timescale may be slightly inaccurate regarding the layer counting between the 1986 and 1963 tie points as it heavily relies on the assumption that the137Cs peak corresponds to the unaltered 1963 signal. This inaccuracy would introduce an additional error of a couple of years.
In Fig. 4c, the SDI data show a distinct impurity peak at 33 m of depth, indicating a layer rich in mineral dust. This layer, found in section 77–78, is approximately 1 m deeper than the main 137Cs peak (section 74–75). The presence of a significant impurity layer beneath the 137Cs peak suggests that the latter is not the result of dust enrichment in a single layer as a result of melting. While impurities can accumulate in a single layer if there is a hidden melting surface within the glacier, potentially leading to radionuclide concentration (Baccolo et al., 2020), the absence of any significant peaks in the SDI dataset at the same depth as the main cesium peak indicates that the cesium peak is not caused by an abundance of dust in this layer. This finding is significant because the cesium data are not calibrated on the amount of dust present but on the volume of filtered water, despite 137Cs being in particulate form. Moreover, the absence of a dust-rich layer at 32 m of depth suggests that even a minimal amount of particulate matter can contribute to a substantial cesium peak.
Our proposed explanation for the lag between the 3H and the 137Cs signals is the presence of meltwater-induced post-depositional processes altering the signal embedded in the ice. 137Cs, mainly bound to particulate matter (Qin et al., 2012; Tanaka et al., 2013; Di Stefano et al., 2019), is more susceptible to percolation than 3H (Pinglot et al., 2003), which is incorporated into water molecules and considered a matrix signal. This result was unexpected as particle-bound signals are generally well preserved within this ice core, leading us to expect similar behavior from cesium. In fact, impurities in particulate matter are usually among the last proxies to be affected by meltwater relocation processes (Meyer and Wania, 2011; Moser et al., 2023). The exact mechanism behind the relocation of cesium is beyond the scope of this study. Further research is needed to investigate the relationship between cesium and the presence of meltwater in ice cores.
Vertical relocation of cesium has been reported in the literature; Pinglot et al. (2003) found a similar delay between cesium and tritium in ice cores extracted from low-altitude glaciers in Svalbard, although the difference between the 3H peak and the 137Cs peak was less pronounced (<1 m). This can be expected since the Adamello Glacier has experienced much more melting than Svalbard, and more time has passed since the deposition of radioactive species onto the glacier. Similarly, Schotterer et al. (1977) reported a high concentration of 137Cs 3 m below the tritium bomb level in an ice core extracted from a relatively low-altitude glacier in the Swiss Alps (Plaine Morte).
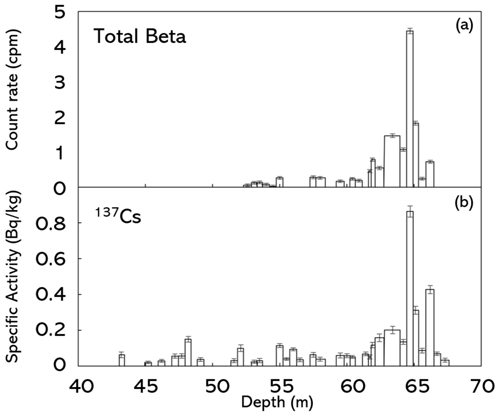
Figure 5Panel (a) shows the total-beta activity from the Lys ice core. Total-beta values are reported here using count rate in terms of counts per minute (cpm) along with the associated uncertainty. Panel (b) shows the specific activity (Bq kg−1) of 137Cs along with the associated error, as reported in Clemenza et al. (2012). In both panels, the width of each bar represents the actual depth covered by each sample.
Our hypothesis is further supported by findings on total-beta measurements that we obtained from an ice core extracted at the Colle del Lys site in 1996 (Smiraglia et al., 2000). Figure 5 shows that in this ice core, the main beta (3H) peak and the main 137Cs peak coincide. Due to its position and high elevation, the Lys Glacier is characterized by a cold regime, as proven by the borehole temperature measurements taken at the time of drilling (Smiraglia et al., 2000). This allows for the radioactivity signal to be preserved in the ice without any relocation. This finding is consistent with another study conducted on an cold Alpine glacier, Grenzgletscher, where the 1963 peaks for beta/tritium and cesium were observed at the same depth (Eichler et al., 2000). The Grenzgletscher and Lys drilling sites are less than 15 km apart.
Kang et al. (2015)Eichler et al. (2000)Schotterer et al. (1977)Table 1Activity values for the 1963 tritium peak at different locations. Data taken from the literature were converted to Bq m−2, considering an ice density of 0.9 g cm−3, and decay corrected to 1963. However, they are to be considered rough approximations. Reported data refer to the single highest sample, if not stated otherwise.
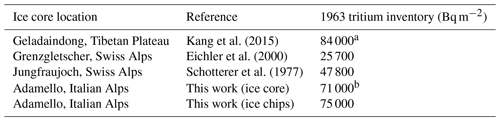
a Sum of three different data points. b Sum of all data points between 30.5 and 31 m.
3.3 Total inventories of 137Cs and 3H
For the Adamello ice core, we calculated the integrated activity of both 137Cs and 3H related to the 1963 fallout events. To do so, data measured in Bq L−1 were converted to Bq m−2, considering the thickness of each sample and an ice density of 0.9 g cm−3. These data were also decay corrected to 1963. For 137Cs, given the signal migration and aiming to be conservative in our calculations, we considered everything below 28 m of depth to be possibly related to the nuclear weapons fallout; taking this into account, we obtained a total inventory of 137Cs amounting to 2597±400 Bq m−2, where the main contribution is given by a single sample (1519±228 Bq m−2). This value is at the lower limit with respect to what is observed in other Alpine glaciers (3500±800 Bq m−2) (Eichler et al., 2000) and in European soils; for example, Bossew et al. (2001) reports a value of 2300 Bq m−2 for 137Cs resulting from bomb fallout in 1986, which corresponds to approximately 3800 Bq m−2 if decay corrected to 1963. These results indicate partial signal loss due to meltwater drainage for the 137Cs signal. Looking at the high-resolution tritium dataset, we calculated a total inventory of 71 000±7000 Bq m−2 for the 1963 event (from 30.5 to 31 m), corrected to 1963, with the peak at 30.5 m corresponding to 7300±700 Bq m−2. For comparison, Oerter and Rauert (1982), in a study of two ice cores extracted from the Vernagtferner glacier (Austrian Alps), which were sampled at a similar thickness resolution to ours, found the highest tritium values, corrected to 1963, to be 4500 and 5300 Bq m−2. In our low-resolution dataset, the single highest sample corresponds to 75 000±5500 Bq m−2. To roughly compare our data to the literature, we converted the data reported in the literature to Bq m−2 and decay corrected them to 1963, as seen in Table 1. Our data exhibit comparable, if not higher, tritium activities than those reported for other ice cores, suggesting that tritium in the Adamello ice core was not lost due to melting, although the region around the main peak appears to be broader than what seen is in the literature, resulting in activity values in terms of Bq L−1 being lower than expected, likely due to diffusion processes.
3.4 Signal preservation in the Adamello ice core
Evidence from the Adamello ice core has shown that proxies in temperate glaciers must be approached with caution. Festi et al. (2021) observed that peaks in pollen concentration and black-carbon concentration, which exhibit exceptionally high values, may represent multiple years condensed into one single layer as a result of negative mass balance years and the enrichment of the impurities at the exposed surface. This poses an issue for annual-layer counting. Despite this, due to the alignment of many palynomorphs and black-carbon peaks, the seasonality of the signal seems to be preserved. In contrast, in Festi et al. (2021), the 210Pb profile did not show a clear exponential decrease in activity concentrations with increasing depth, as typically observed in glacier ice. 210Pb concentrations in the literature have shown large fluctuations connected with dirt horizons (Gaggeler et al., 1983), indicating that 210Pb may be transported with water, thus preventing the meaningful dating of temperate glaciers with this nuclide. 210Pb shows behavior in ice that is similar to what is observed for cesium: these highly insoluble elements remain bound to particulate matter and are prone to relocation and loss of signal when meltwater is present. Unexpectedly, in this ice core, the cesium peak was not coincident with a dust layer. Furthermore, by comparing the highest 137Cs peak to activity levels attributed to 1963 found at the Colle Gnifetti site (Eichler et al., 2000), we observed a loss of the expected signal of more than 50 %. However, when examining tritium, the record appears to be mostly preserved – at least for the post-1960s period (as the 1954 and 1958 peaks were not detected in this ice core). This conclusion has been drawn from the shape of the tritium profile matching the tritium precipitation record and from comparing the total inventory, where no loss in tritium activity has been found. This provides further confirmation of the better preservation of tritium compared to that of cesium. We thus believe that the Adamello Glacier can still function as a paleoclimate archive. However, to correctly interpret the information contained therein, it is necessary to consider the melting and disturbance processes affecting the climatic signals.
Using liquid scintillation counting, we analyzed tritium in the entire 2016 Adamello ice core with a resolution of approximately 0.5 m and an increased resolution for the portion between 27 and 35 m of depth. Our analysis showed contamination of tritium between 19 and 32 m of depth, attributed to the worldwide radioactive contamination caused by atmospheric nuclear-bomb testing in the 1950s and 1960s. Additionally, we were able to compare the 3H profile with another byproduct of nuclear-bomb testing, 137Cs. The analysis revealed that for the two artificial radionuclides, the radioactive peak associated with 1963 is not coincident. The 3H peak occurs 1.5 m above the 137Cs one. As many records show that the deposition of the two radioactive species was concurrent, the shift in the peaks is only explainable by assuming that the 137Cs peak has been subject to downward relocation triggered by meltwater percolation in temperate ice. Tritium was less affected by the process as it is present in the ice matrix. On the contrary, 137Cs, as it is more soluble and mobile in aqueous environments, is more prone to relocation if liquid water is present. While the total tritium inventory associated with the 1963 peak in the Adamello ice core generally agrees with previous findings for cold glaciers that are not affected by melting, we find that this is not the case for 137Cs. The total inventory of 137Cs calculated at the 1963 peak for this radionuclide is among the lowest ever reported, providing additional evidence that the position of cesium within the ice was disrupted by meltwater, leading also to partial removal through washing. The results in this study indicate that there are potential issues with the established application of radionuclides for dating mountain ice cores. While for a cold glacier, it is reasonable to assume that radioactive signals are well preserved in the ice column, for temperate ice cores, this is not the case. To avoid potential dating inaccuracies, it is preferable to reconstruct the entire profile of 3H to obtain a stronger indication of the true 1963 signal depth. In general, when disturbances are present in the reconstructed profile, matrix signals (such as those regarding tritium) are more easily preserved. Additional studies are needed to investigate the relocation mechanism of cesium in the presence of meltwater.
All data are available upon request to the authors.
EDS and MC conceived the idea for this work. EDS carried out the sample preparation for liquid scintillation counting. DF and RG acquired and analyzed the hyperspectral data. EDS, GB, and BD interpreted the data. EDS prepared the paper with contributions from all co-authors. MS and VM provided additional editing and contributed to the review process.
The contact author has declared that none of the authors has any competing interests.
Publisher's note: Copernicus Publications remains neutral with regard to jurisdictional claims made in the text, published maps, institutional affiliations, or any other geographical representation in this paper. While Copernicus Publications makes every effort to include appropriate place names, the final responsibility lies with the authors.
This work is a contribution to the project “CALICE: Calibrating Plant Biodiversity in Glacier Ice”, a multidisciplinary program involving the University of Innsbruck, the Free University of Bozen-Bolzano, and the Fondazione Edmund Mach in San Michele all'Adige, funded by the EVTZ/Austrian Science Fund (grant no. IPN 57-B22). This is the second publication of the CALICE project. We would like to thank all members of the CALICE scientific consortium, especially those who helped during the coring activities and the processing of the ice core. We are grateful to the ENEA drilling team and the Alpine guide Nicola Viotti (Guide Alpine Valsusa) for their excellent work during the coring campaign. Drilling has been possible thanks to a specific grant (POLLiCE) awarded to the FEM (Fondazione Edmund Mach) from the Autonomous Province of Trento (PAT) and logistical support (helicopter flights) provided by Ernesto Sanutuliana. EuroCold laboratory activities were partially funded by the Department for Regional Affairs and Autonomies (grant no. 2014-CONV-0032).
This research has been supported by the Austrian Science Fund (grant no. IPN 57-B22) and the Department for Regional Affairs and Autonomies (grant no. 2014-CONV-0032).
This paper was edited by Valentina Radic and reviewed by four anonymous referees.
Avak, S. E., Schwikowski, M., and Eichler, A.: Impact and implications of meltwater percolation on trace element records observed in a high-Alpine ice core, J. Glaciol., 64, 877–886, 2018. a
Baccolo, G., Łokas, E., Gaca, P., Massabò, D., Ambrosini, R., Azzoni, R. S., Clason, C., Di Mauro, B., Franzetti, A., Nastasi, M., Prata, M., Prati, P., Previtali, E., Delmonte, B., and Maggi, V.: Cryoconite: an efficient accumulator of radioactive fallout in glacial environments, The Cryosphere, 14, 657–672, https://doi.org/10.5194/tc-14-657-2020, 2020. a
Bossew, P., Ditto, M., Falkner, T., Kienzl, K., and Rappelsberger, U.: Contamination of Austrian soil with caesium-137, J. Environ. Radioact., 55, 187–194, 2001. a
Clemenza, M., Cucciati, G., Maggi, V., Pattavina, L., and Previtali, E.: Radioactive fallouts as temporal makers for glacier ice cores dating, Eur. Phys. J. Plus, 127, 1–8, 2012. a, b, c
Currie, L. A.: Limits for qualitative detection and quantitative determination. Application to radiochemistry, Anal. Chem., 40, 586–593, 1968. a, b
Davaze, L., Rabatel, A., Dufour, A., Hugonnet, R., Arnaud, Y., and Braithwaite, R. J.: Region-Wide Annual Glacier Surface Mass Balance for the European Alps From 2000 to 2016, Front. Earth Sci., 8, 1–14, https://doi.org/10.3389/feart.2020.00149, 2020. a
Di Mauro, B., Fava, F., Ferrero, L., Garzonio, R., Baccolo, G., Delmonte, B., and Colombo, R.: Mineral dust impact on snow radiative properties in the European Alps combining ground, UAV, and satellite observations, J. Geophys. Res., 120, 6080–6097, https://doi.org/10.1002/2015JD023287, 2015. a
Di Stefano, E., Clemenza, M., Baccolo, G., Delmonte, B., and Maggi, V.: 137Cs contamination in the Adamello glacier: Improving the analytical method, J. Environ. Radioact., 208–209, 106039, https://doi.org/10.1016/j.jenvrad.2019.106039, 2019. a, b, c, d, e
Eichler, A., Schwikowski, M., Gäggeler, H. W., Furrer, V., Synal, H.-A., Beer, J., Saurer, M., and Funk, M.: Glaciochemical dating of an ice core from upper Grenzgletscher (4200 m asl), J. Glaciol., 46, 507–515, 2000. a, b, c, d, e, f, g
Eichler, A., Schwikowski, M., and Gäggeler, H. W.: Meltwater-induced relocation of chemical species in Alpine firn, Tellus B, 53, 192–203, https://doi.org/10.3402/tellusb.v53i2.16575, 2001. a
Festi, D., Schwikowski, M., Maggi, V., Oeggl, K., and Jenk, T. M.: Significant mass loss in the accumulation area of the Adamello glacier indicated by the chronology of a 46 m ice core, The Cryosphere, 15, 4135–4143, https://doi.org/10.5194/tc-15-4135-2021, 2021. a, b, c, d, e, f
Gabrieli, J., Cozzi, G., Vallelonga, P., Schwikowski, M., Sigl, M., Eickenberg, J., Wacker, L., Boutron, C., Gäggeler, H., Cescon, P., and Barbante, C.: Contamination of Alpine snow and ice at Colle Gnifetti, Swiss / Italian Alps, from nuclear weapons tests, Atmos. Environ., 45, 587–593, https://doi.org/10.1016/j.atmosenv.2010.10.039, 2011. a
Gabrielli, P., Carturan, L., Gabrieli, J., Dinale, R., Krainer, K., Hausmann, H., Davis, M., Zagorodnov, V., Seppi, R., Barbante, C., Fontana, G. D., and Thompson, L. G.: Atmospheric warming threatens the untapped glacial archive of Ortles mountain, South Tyrol, J. Glaciol., 56, 843–853, https://doi.org/10.3189/002214310794457263, 2010. a
Gaggeler, H., Von Gunten, H. R., Rossler, E., Oeschger, H., and Schotterer, U.: 210Pb dating of cold alpine firn/ ice cores from Colle Gnifetti, Switzerland., J. Glaciol., 29, 165–177, https://doi.org/10.1017/S0022143000005220, 1983. a
Garzonio, R., Di Mauro, B., Cogliati, S., Rossini, M., Panigada, C., Delmonte, B., Maggi, V., and Colombo, R.: A novel hyperspectral system for high resolution imaging of ice cores: Application to light-absorbing impurities and ice structure, Cold Reg. Sci. Technol., 155, 47–57, https://doi.org/10.1016/j.coldregions.2018.07.005, 2018. a
Gilbert, A., Wagnon, P., Vincent, C., Ginot, P., and Funk, M.: Atmospheric warming at a high-elevation tropical site revealed by englacial temperatures at Illimani, Bolivia (6340 m above sea level, 16° S, 67° W), J. Geophys. Res.-Atmos., 115, 1–10, https://doi.org/10.1029/2009JD012961, 2010. a
Gilbert, A., Sinisalo, A., Gurung, T. R., Fujita, K., Maharjan, S. B., Sherpa, T. C., and Fukuda, T.: The influence of water percolation through crevasses on the thermal regime of a Himalayan mountain glacier, The Cryosphere, 14, 1273–1288, https://doi.org/10.5194/tc-14-1273-2020, 2020. a
Harms, P. A., Visser, A., Moran, J. E., and Esser, B. K.: Distribution of tritium in precipitation and surface water in California, J. Hydrol., 534, 63–72, https://doi.org/10.1016/j.jhydrol.2015.12.046, 2016. a
Hock, R., Bliss, A., Marzeion, B. E. N., Giesen, R. H., Hirabayashi, Y., Huss, M., Radić, V., and Slangen, A. B.: GlacierMIP – A model intercomparison of global-scale glacier mass-balance models and projections, J. Glaciol., 65, 453–467, https://doi.org/10.1017/jog.2019.22, 2019. a
Hoelzle, M., Darms, G., Lüthi, M. P., and Suter, S.: Evidence of accelerated englacial warming in the Monte Rosa area, Switzerland/Italy, The Cryosphere, 5, 231–243, https://doi.org/10.5194/tc-5-231-2011, 2011. a
International Atomic Energy Agency: Environmental Consequences of the Chernobyl Accident and their Remediation: Twenty years of Experience, Tech. rep., https://doi.org/10.1007/bf02850918, 2006. a
International Atomic Energy Agency and World Meteorological Organization: Global Network of Isotopes in Precipitation, The GNIP Database, https://nucleus.iaea.org/wiser (last access: May 2024), 2021. a, b
Jouzel, J., Merlivat, L., Pourchet, M., and Lorius, C.: A Countinuous Record of Artificial Tritium Fallout at the South Pole (1954–1978), Earth Planet. Sc. Lett., 45, 188–200, 1979. a
Kang, S., Wang, F., Morgenstern, U., Zhang, Y., Grigholm, B., Kaspari, S., Schwikowski, M., Ren, J., Yao, T., Qin, D., and Mayewski, P. A.: Dramatic loss of glacier accumulation area on the Tibetan Plateau revealed by ice core tritium and mercury records, The Cryosphere, 9, 1213–1222, https://doi.org/10.5194/tc-9-1213-2015, 2015. a, b, c
Kaufman, S. and Libby, W. F.: The natural distribution of tritium, Phys. Rev., 93, 1337–1344, https://doi.org/10.1103/PhysRev.93.1337, 1954. a
Lliboutry, L.: Permeability, Brine Content and Temperature of Temperate Ice, J. Glaciol., 10, 15–29, https://doi.org/10.3189/s002214300001296x, 1971. a
Lucas, L. L. and Unterweger, M. P.: Comprehensive Review and Critical Evaluation, Journal of research of the National Institute of Standards and Technology, 105, 541–549, 2000. a
Masarik, J. and Beer, J.: An updated simulation of particle fluxes and cosmogenic nuclide production in the Earth's atmosphere, J. Geophys. Res.-Atmos., 114, 1–9, https://doi.org/10.1029/2008JD010557, 2009. a
Meyer, T. and Wania, F.: Modeling the elution of organic chemicals from a melting homogeneous snow pack, Water Res., 45, 3627–3637, https://doi.org/10.1016/j.watres.2011.04.011, 2011. a
Michel, R. L.: Tritium in the hydrologic cycle, in: Isot. Water Cycle Past, Present Futur. a Dev. Sci., 53–66, ISBN 140203010X, https://doi.org/10.1007/1-4020-3023-1_5, 2005. a
Mikhalenko, V., Sokratov, S., Kutuzov, S., Ginot, P., Legrand, M., Preunkert, S., Lavrentiev, I., Kozachek, A., Ekaykin, A., Faïn, X., Lim, S., Schotterer, U., Lipenkov, V., and Toropov, P.: Investigation of a deep ice core from the Elbrus western plateau, the Caucasus, Russia, The Cryosphere, 9, 2253–2270, https://doi.org/10.5194/tc-9-2253-2015, 2015. a, b
Moser, D. E., Thomas, E. R., Nehrbass-Ahles, C., Eichler, A., and Wolff, E.: Review article: Melt-Affected Ice Cores for (Sub-)Polar Research in a Warming World, EGUsphere [preprint], https://doi.org/10.5194/egusphere-2023-1939, 2023. a, b
Niu, H., Kang, S., Shi, X., Paudyal, R., He, Y., Li, G., Wang, S., Pu, T., and Shi, X.: In-situ measurements of light-absorbing impurities in snow of glacier on Mt. Yulong and implications for radiative forcing estimates, Sci. Total Environ., 581–582, 848–856, https://doi.org/10.1016/j.scitotenv.2017.01.032, 2017. a
Oerter, H. and Rauert, W.: Core drilling on Vernagtferner ( Oetztal Alps, Austria) in 1979: tritium contents., Zeitschrift fur Gletscherkd. und Glazialgeol., 18, 13–22, 1982. a, b, c
Pavlova, P. A., Jenk, T. M., Schmid, P., Bogdal, C., Steinlin, C., and Schwikowski, M.: Polychlorinated biphenyls in a temperate alpine glacier: 1. Effect of percolating meltwater on their distribution in glacier ice, Environ. Sci. Technol., 49, 14085–14091, 2015. a, b
Picciotto, E. and Wilgain, S.: Fission Products in Antarctic Snow, A Reference Level for Measuring Accumulation, J. Geophys. Res., 68, 5965–5972, 1963. a
Picotti, S., Francese, R., Giorgi, M., Pettenati, F., and Carcione, J. M.: Estimation of glacier thicknesses and basal properties using the horizontal-to-vertical component spectral ratio (HVSR) technique from passive seismic data, J. Glaciol., 63, 229–248, https://doi.org/10.1017/jog.2016.135, 2017. a
Pinglot, J. F., Vaikmäe, R. A., Kamiyama, K., Igarashi, M., Fritzsche, D., Wilhelms, F., Koerner, R., Henderson, L., Isaksson, E., Winther, J.-G., Van de Wal, R. S. W., Fournier, M., Bouisset, P., and Meijer, H. A. J.: Ice cores from Arctic sub-polar glaciers: chronology and post-depositional processes deduced from radioactivity measurements, J. Glaciol., 49, 149–158, 2003. a, b, c
Pourchet, M. and Pinglot, F.: Determination of the stratospheric residence time from the total beta activity of Antarctic and Greenland snows, Geophys. Res. Lett., 6, 6–8, 1979. a
Qiao, J., Colgan, W., Jakobs, G., and Nielsen, S.: High-Resolution Tritium Pro fi le in an Ice Core from Camp Century, Greenland, Environ. Sci. Technol., 55, 13638–13645, https://doi.org/10.1021/acs.est.1c01975, 2021. a
Qin, H., Yokoyama, Y., Fan, Q., Iwatani, H., Tanaka, K., Sakaguchi, A., Kanai, Y., Zhu, J., Onda, Y., and Takahashi, Y.: Investigation of cesium adsorption on soil and sediment samples from Fukushima prefecture by sequential extraction and EXAFS technique, Geochem. J., 46, 297–302, https://doi.org/10.2343/geochemj.2.0214, 2012. a
Ranzi, R., Grossi, G., Gitti, A., and Taschner, S.: Energy and mass balance of the mandrone glacier (Adamello, Central Alps), Geogr. Fis. e Din. Quat., 33, 45–60, 2010. a
Schotterer, U., Finkel, R. C., Oeschger, H., Wahlen, M., Bart, G., and Gäggeler, H. W.: Isotope measurements on firn and ice cores from alpine glaciers, IAHS, 232–236, 1977. a, b, c
Schotterer, U., Schwarz, P., and Rajner, V.: FROM PRE-BOMB LEVELS TO INDUSTRIAL TIMES A complete tritium record from an alpine ice core and its relevance for environmental studies, ISBN 9201005989, 1998a. a
Schotterer, U., Schwarz, P., and Rajner, V.: From pre-bomb levels to industrial times: A complete tritium record from an alpine ice core and its relevance for environmental studies, Isot. Tech. study Environ. Chang., International Atomic Energy Agency, Vienna (Austria), 932 pp., ISBN 92-0-100598-9, International symposium on isotope techniques in the study of past and current environmental changes in the hydrosphere and the atmosphere, Vienna (Austria); 14–18 April 1997, IAEA-SM–349/55, 1998b. a, b
Schwikowski, M., Briitsch, S., G, H. W., Schotterer, U., and Scherrer, P.: A high-resolution air chemistry record from an Alpine ice core: Fiescherhorn glacier, Swiss Alps suitable glaciers are often small in the horizontal and hindered by atmospheric stability [Baltensperger extension, thus limiting the accessible timescale], Europe, 104, 709–719, 1999. a
Smiraglia, C., Maggi, V., Novo, A., Rossi, G., Johnston, P., Sr, A., Maggi, V., Gc, R., and Johnston, P.: Premilinary Results of Two Ice Core Drillings on Monte Rosa (Colle Gnifetti and Colle del Lys), Italian Alps, Geogr. Fis. e Din. Quat., 165–172, 2000. a, b, c
Steinhauser, G., Brandl, A., and Johnson, T. E.: Comparison of the Chernobyl and Fukushima nuclear accidents: A review of the environmental impacts, Sci. Total Environ., 470–471, 800–817, https://doi.org/10.1016/j.scitotenv.2013.10.029, 2014. a
Tanaka, K., Sakaguchi, A., Kanai, Y., Tsuruta, H., Shinohara, A., and Takahashi, Y.: Heterogeneous distribution of radiocesium in aerosols, soil and particulate matters emitted by the Fukushima Daiichi Nuclear Power Plant accident: Retention of micro-scale heterogeneity during the migration of radiocesium from the air into ground and rive, J. Radioanal. Nucl. Chem., 295, 1927–1937, https://doi.org/10.1007/s10967-012-2160-9, 2013. a
Thatcher, L. L.: The distribution of tritium fallout in precipitation over north america, Int. Assoc. Sci. Hydrol. Bull., 7, 48–58, https://doi.org/10.1080/02626666209493255, 1962. a
Thompson, L. G., Mosley-Thompson, E., Davis, M., Lin, P. N., Yao, T., Dyurgerov, M., and Dai, J.: “Recent warming”: ice core evidence from tropical ice cores with emphasis on Central Asia, Glob. Planet. Change, 7, 145–156, https://doi.org/10.1016/0921-8181(93)90046-Q, 1993. a
United Nations Scientific Committee on the Effects of Atomic Radiation: Sources and Effects of Ionizing R adiation, Tech. rep., ISBN 9211422388, 2000. a
United Nations Scientific Committee on the Effects of Atomic Radiation: Sources And Effects of Ionizing Radiation, Tech. Rep. 4, ISBN 9789211422740, https://doi.org/10.1093/oxfordjournals.rpd.a079988, 2008. a
Villa, S., Negrelli, C., Maggi, V., Finizio, A., and Vighi, M.: Analysis of a firn core for assessing POP seasonal accumulation on an Alpine glacier, Ecotoxicol. Environ. Saf., 63, 17–24, https://doi.org/10.1016/j.ecoenv.2005.05.006, 2006. a
Wagenbach, D., Bohleber, P., Preunkert, S., Wagenbach, D., Bohleber, P., and Preunkert, S.: Cold , alpine ice bodies revisited : what may we learn from their impurity and isotope content?, Geogr. Ann. Ser. A, 94, 245–263, https://doi.org/10.1111/j.1468-0459.2012.00461.x, 2012. a
Wong, G. J., Hawley, R. L., Lutz, E. R., and Osterberg, E. C.: Trace-element and physical response to melt percolation in Summit (Greenland) snow, Ann. Glaciol., 54, 52–62, https://doi.org/10.3189/2013AoG63A602, 2013. a
Yasunari, T. J., Shiraiwa, T., Kanamori, S., Fujii, Y., Igarashi, M., Yamazaki, K., Benson, C. S., and Hondoh, T.: Intra-annual variations in atmospheric dust and tritium in the North Pacific region detected from an ice core from Mount Wrangell, Alaska, J. Geophys. Res.-Atmos., 112, 1–16, https://doi.org/10.1029/2006JD008121, 2007. a
Zebre, M., Colucci, R. R., Giorgi, F., Glasser, N. F., Racoviteanu, A. E., and Del Gobbo, C.: 200 years of equilibrium-line altitude variability across the European Alps (1901–2100), Clim. Dynam., 56, 1183–1201, https://doi.org/10.1007/s00382-020-05525-7, 2021. a
Zemp, M., Huss, M., Thibert, E., Eckert, N., Mcnabb, R., Huber, J., Barandun, M., and Machguth, H.: Global glacier mass changes and their contributions to sea-level rise from 1961 to 2016, Nature, 568, 382–386, 2019. a