the Creative Commons Attribution 4.0 License.
the Creative Commons Attribution 4.0 License.
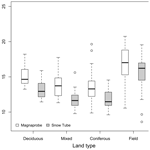
Brief communication: Comparison of in situ ephemeral snow depth measurements over a mixed-use temperate forest landscape
Holly Proulx
Jennifer M. Jacobs
Elizabeth A. Burakowski
Eunsang Cho
Adam G. Hunsaker
Franklin B. Sullivan
Michael Palace
Cameron Wagner
The accuracy and precision of snow depth measurements depend on the measuring device and the conditions of the site and snowpack in which it is being used. This study compares collocated snow depth measurements from a magnaprobe snow depth probe and a Federal snow tube in an ephemeral snow environment. We conducted three snow depth sampling campaigns from December 2020 to February 2021 that included 39 open-field and coniferous-, mixed-, and deciduous-forest sampling sites in Durham, New Hampshire, United States. For all sampling campaigns and land cover types, with a total of 936 paired observations, the magnaprobe snow depth measurements were consistently deeper than those of the snow tube. There was a 12 % average difference between the magnaprobe (14.9 cm) and snow tube (13.2 cm) average snow depths with a greater difference in the forest (1.9 cm) than the field (1.3 cm). This study suggests that snow depth measurements using a Federal snow tube can avoid overprobing with an ephemeral snowpack in forested environments.
- Article
(2691 KB) - Full-text XML
-
Supplement
(392 KB) - BibTeX
- EndNote
Snow depth is one of the easier snowpack properties to measure in the field and is an observation that can be measured relatively precisely without considerable expertise or expense. Hundreds of snow depth measurements can readily be taken in a single day, and automated samplers can substantially increase that number (Sturm and Holmgren, 2018). In situ snow depth observations can be measured manually or automatically. While automated measurements are increasing in terms of use (Bongio et al., 2021; Kinar and Pomeroy, 2015; Kopp et al., 2019), in situ measurements remain the mainstay of data collection research and operations (Kinar and Pomeroy, 2015; Pirazzini et al., 2018). Manual in situ snow depth measurements are typically made using snow stakes, rulers, or narrow-diameter snow probes (Kinar and Pomeroy, 2015; Pirazzini et al., 2018). Snow tube samplers, which have been in use since the 1930s, also measure snow depth. The magnaprobe, an automatic snow depth probe that records snow depth and GPS measurements, has considerably increased the number of georeferenced snow depth observations that can be made in a single day and is used extensively for snow depth research campaigns (Sturm and Holmgren, 2018; Walker et al., 2020). Measurement variability and errors are sometimes reduced by repeating the measurement, typically three times (Leppänen et al., 2016). Because snow depth is assumed to have greater spatial variability than snow density (Elder et al., 1998), a snow survey often makes numerous snow depth measurements per snow density measurement and then combines these to obtain the snow water equivalent (SWE) (López-Moreno et al., 2013). If depth can be well constrained, then density becomes the source of uncertainty (Raleigh and Small, 2017).
SWE measurement errors associated with snow tube samplers are relatively well understood and characterized. Known issues include biases as compared to snow pit measurements (Dixon and Boon, 2012; Farnes et al., 1983; Goodison et al., 1978; Sturm et al., 2010), accuracies around ±5 % to 10 % for an individual instrument, and differences among SWEs from different snow tube models (e.g., the Meteorological Service of Canada, the Federal or Mt. Rose, the Adirondack, and the Snow-Hydro) that can exceed 10 % (Farnes et al., 1983). Less is understood about the errors in snow depth measurements. López-Moreno et al.'s (2020) comparison of nine snow core samplers found that snow depths were relatively consistent when taken over a paved surface. However, over uneven ground, the snow depth differences among samplers were much greater, and replicated snow depth measurements had larger variability as compared to the snow density. The magnaprobe, which measures snow depth with a precision of less than 0.1 mm, has the potential for low biases if its basket settles into soft surface snow, but those biases are typically less than 1 cm (Sturm and Holmgren, 2018). When the rod penetrates the substrate (overprobing), the error depends on the ground surface and the operation. Solid or frozen ground surfaces have negligible overprobing, but unfrozen natural surfaces may have considerable penetration (Derry et al., 2009) with biases on the order of 5 to 10 cm (Berezovskaya and Kane, 2007; Sturm and Holmgren, 2018). These errors can have profound effects on SWE estimates in shallow snow environments and represent a challenge for error accounting in hydrological modeling.
The goal of this brief study is to determine (1) if the magnitudes of the snow depth measurements using a magnaprobe and a Federal tube are significantly different in an ephemeral snow environment with shallow snow and (2) if the differences vary by land cover type. We hypothesize that the snow depth measurements from the magnaprobe will be deeper than those from the snow tube. This hypothesis is based on the understood errors and biases associated with the magnaprobe and the Federal tube, including the smaller surface area of the probe, which allows for greater penetration through snowpacks and leaf litter. Three snow depth sampling campaigns were conducted from December 2020 to March 2021 over field and forest plots at Thompson Farm in Durham, New Hampshire, USA.
2.1 Study site
This study was conducted at the University of New Hampshire's Thompson Farm Earth Systems Observatory in southeast New Hampshire, United States (43.11∘ N, 70.95∘ W; 35 m above sea level, a.s.l.). The site has mixed hardwood-forest and open-field land covers (Perron et al., 2004) that are characteristic of the region (Fig. 1). The agricultural fields are managed pasture grass with unmown grass in local areas. The deciduous, mixed, and coniferous forest is composed primarily of white pine (Pinus strobus), northern red oak (Quercus rubra), red maple (Acer rubrum), shagbark hickory (Carya ovata), and white oak (Quercus alba) (Perron et al., 2004). The forest soils are classified as Hollis–Charlton very stony fine sandy loam and are well drained; field soils are characterized as Scantic silt loam and are poorly drained.
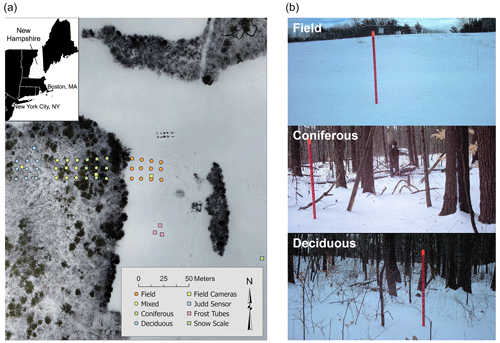
Figure 1(a) The 4 February 2021 aerial optical image of Thompson Farm, Durham NH, USA, showing both forest and field regions with snow sampling sites in the field and in the coniferous-, mixed-, and deciduous-forested areas, as well as the locations of the soil frost tubes and field cameras. (b) Field camera images in the field and in the coniferous and deciduous forested areas taken on 4 February 2021 by the field cameras.
In situ sampling was conducted at 39 sites located along three parallel transects (Fig. 1). The approximately 145 m long transects were laid out from east to west. The transects were separated by approximately 10 m, north to south. From east to west, each transect started in the open-field area and then transitioned to the coniferous-, the mixed-, and finally the deciduous-forested areas. Each of the three transects had 13 sampling sites; 4 sites were in the open-field area, 3 were in the coniferous forest, 3 were in the mixed forest, and 3 were in the deciduous forest. These were all marked with a stake. The stake locations were geolocated using a Trimble© Ge 7X GNSS positioning unit and Zephyr™ antenna with an estimated horizontal uncertainty of 2.51 cm (standard deviation of 0.95 cm) and 4.17 cm (standard deviation of 4.60 cm) for the field and forest, respectively, after differential correction. Three soil frost tubes were located in the field approximately 25 m south of the field transect, and another three were located in the forest about 100 m southwest of the study area.
2.2 In situ sampling methods
Snow depth was measured using a magnaprobe and a Federal snow sampler, also known as a snow tube. The Federal snow tube, with its long operational history (Clyde, 1932), served as a historical reference against the magnaprobe. A magnaprobe consists of an avalanche probe-like rod of about 1.5 m in length that contains a magnetostrictive device and a sliding magnetic disk-shaped basket with a 25 cm diameter. The rod has a 1.27 cm diameter with an affixed tip that tapers to a point to help penetrate ice layers. The magnaprobe was operated by inserting the pole into a snowpack until the tip of the pole reached the ground surface, allowing the basket to slide down to float on top of the snow. A handheld portable keypad connected to a data logger recorded the snow depth between the tip of the pole and the bottom of the basket.
A Federal snow sampler is an aluminum tube, about 76 cm in length and with a 4.13 cm inner diameter, that is used to measure snow depth and SWE (Clyde, 1932). To measure snow depth, the snow tube was inserted vertically into the snowpack until it reached the ground, and a depth was read at eye level. Snow depth was recorded to the nearest 0.5 cm. To measure snow density, the snow tube was then lifted out of the snowpack using a spatula as needed to ensure that snow did not fall out of the tube. The snow and snow tube were weighed using a digital hanging scale (CCi HS-6 Electronic Scale, 2 g resolution).
Sampling campaigns were conducted on 18 December 2020, 4 February 2021, and 24 February 2021. A total of 936 paired magnaprobe and Federal snow tube snow depth observations were collected during the three sampling campaigns. At each of the 39 sampling locations, nine measurements were made in a 1 × 1 m area. At each location, a 1 × 1 m square polyvinyl chloride (PVC) grid was placed on the snow surface with one vertex located coincident with a stake. The orientation of two adjacent sides of the grid was recorded using a compass. Nine magnaprobe depth measurements were made at approximately even spacing within the grid. Immediately after the magnaprobe measurements, snow tube snow depth measurements were made at the same nine locations by positioning the snow tube directly over each magnaprobe sampling location. At a 10th location within each 1 × 1 m grid, the snow tube was used to make a snow density measurement. For the 24 February 2021 campaign, after the magnaprobe measurements were completed for the two northern transects, the instrument was transferred to a new operator, who made measurements on the southernmost transect (Transect 1). Transect 1 data for that date were removed from the analysis because the quality assurance and quality control (QA-QC) process identified notable errors for observations from that transect.
Moultrie Wingscapes BirdCam Pro field cameras were used to capture images of the snowpack every 15 min relative to a 1.5 m marked PVC pole following the method used in NASA's 2020 SnowEx field camera campaign in Grand Mesa, CO (Chris Heimstra, personal communication, 16 November 2020). Three cameras were used; one was in the open field, one was in the coniferous forest, and one was in the deciduous forest (Fig. 1). Snow depth was derived by manual inspection of the photos and was recorded to the nearest centimeter.
2.3 Ancillary soils and vegetation cover data
Daily soil frost depth data were collected at field and forest locations at the Thompson Farm Earth Systems Observatory using frost tubes in the style of the Cold Regions Research and Engineering Laboratory (Gandahl, 1957). The frost tubes have flexible, polyethylene inner tubing filled with methylene blue dye, whose color change is easy to differentiate when extruded from ice. The outer tubing consists of PVC pipe installed between 0.4 and 0.5 m below the soil surface. The field and forest sites each had three soil frost tubes.
Leaf litter depth was measured on 2 April 2021 after the spring snowmelt. The leaf litter depth was measured at each snow depth sample location. Sampling was conducted using a PVC collar or round ring that was 8 cm in depth and 10 cm in diameter (Kaspari and Yanoviak, 2008). The collar was placed in the leaf litter and was pushed down until it was through the leaf litter layer. If sticks or larger stones were in the way, they were carefully removed, or the collar was moved slightly to an adjacent location. Measurements were taken using a wooden ruler at four cardinal points in the collar. The four measurements were recorded, and their average to the nearest centimeter was used as the final litter depth. The range of leaf litter depths measured in the forest using the collar was typically 3 to 7 cm, with an average leaf litter depth of 3.9 cm. Magnaprobe leaf litter penetration depth measurements, also made on 2 April 2021 in the forest, had an average value of 5.8 cm.
The three sampling campaigns (18 December 2020, 4 February 2021, and 24 February 2021) all had shallow snowpacks. The field camera observations indicate that the snowpacks had similar depths, between 10 and 15 cm, on the three sampling dates, with modestly deeper snow in the field than the forest. The deepest snow was found on 4 February 2021 with 15 cm in the field and 9.3 cm in the forest. Between the 18 December and 4 February sampling campaigns, there was a melt event in which the entire 10 cm snowpack found on 18 December ablated. The next significant snowfall event (15 cm) occurred on 1 February 2021. The snowpack experienced little additional accumulation or ablation between 4 February and 24 February. The 4 February (0.15 g cm−3) and 24 February (0.20–0.24 g cm−3) snowpack density values were higher than those for December (∼ 0.10 g cm−3). There were shallow soil frost depths (<4 cm) during the early-winter 18 December campaign in the forest and the field. Deeper soil frost depths of 15.1 cm in the field and 5.9 cm in the forest occurred on 4 February 2021, with similar soil frost conditions on 24 February 2021.
3.1 Magnaprobe vs. snow tube
The full experiment yielded 936 individual pairs of snow depth measurements from the snow tube and the magnaprobe (Fig. 2a). For the comparison between measurement techniques, the orthogonal Deming regression method was applied to consider measurement errors in both variables. Overall, there was moderate agreement (R=0.74) between the two datasets for all three sampling campaigns (Table S1 in the Supplement). The snow depths measured by the magnaprobe (14.9 cm average snow depth) were deeper than the snow tube (13.2 cm average snow depth) with an overall bias of 1.7 cm. The magnaprobe snow depth was at least 0.5 cm deeper than the snow tube in 74 % of the 936 measurement pairs. Only 6.3 % of the pairs had snow tube snow depths exceeding magnaprobe snow depths by 0.5 cm or more. Conversely, 7.4 % of the pairs' magnaprobe snow depths were over 5.0 cm deeper than the snow tube. In eight pairs of measurements, when the magnaprobe measured snow depths greater than 15 cm, the magnaprobe snow depths were more than double the snow tube snow depths.
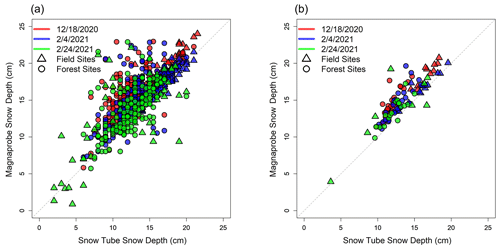
Figure 2Comparison of snow depths measured by magnaprobe and snow tube for the three sampling campaigns using (a) the individual sampling points (n=936) and (b) grid cell average values (n=104). The date format in the figure is month/day/year.
The majority of the nine sampling locations in each grid had magnaprobe snow depth values that were deeper than those measured using the snow tube. For all the grids, an average of 8.7, 7.7, and 7.0 out of the 9 sampling locations had deeper magnaprobe snow depths on 18 December 2020, 4 February 2021 and 24 February 2021, respectively. As hypothesized, t-test results showed that the magnaprobe snow depth values were significantly greater than those measured using the snow tube for 39 and 31 of the 39 sampling locations on 18 December 2020, and 4 February 2021, respectively, but only 11 out of the 26 sampling locations on 24 February 2021. The mean differences were 2.3, 1.4, and 1.6 cm, with root mean square difference (RMSD) values of 3.0, 2.3, and 3.3 cm on 18 December 2020, 4 February 2021, and 24 February 2021, respectively, which is on the order of 15 % to 25 % of the overall depth observed during these campaigns. Despite the biases, the average within-cell snow depth variability was nearly identical for the magnaprobe and the snow tube in the field (1.3 cm standard deviation for the magnaprobe). In the forest, the magnaprobe's 2.0 cm within-cell standard deviation modestly exceeded the snow tube's 1.5 cm standard deviation. A slightly reduced agreement was found on 24 February when there was a 1 to 4 cm thick ice layer at the bottom of the snowpack in local depressions.
The overall agreement between the snow tube and magnaprobe was better when the nine measurements within a single 1 × 1 m grid cell were averaged at each of the sampling locations (Fig. 2b and Table S1). There is a notable improvement in grid cell statistics, and the correlation is stronger (overall R=0.87), with slopes closer to 1, intercepts closer to 0, and RMSD values reduced to 2.5 cm or less. Although averaging has no impact on the overall bias, the range of differences among pairs narrowed. The difference between the magnaprobe and the snow tube is typically constrained to less than 3 cm with a limited number of outliers. The magnaprobe snow depth was at least 0.5 cm deeper than the snow tube in almost all grid cells (86.7 %), but only three grid cells had differences greater than 5 cm. Among the grid-averaged magnaprobe snow depths, there were no instances in which there was a doubling of snow depth when compared to the snow tube measurements.
3.2 Magnaprobe vs. snow tube by land type
The magnaprobe and snow tube snow depths differ by land type, with the field having deeper snow and more spatial variability than the forest land types (Fig. 3). Among the three forest types, the deepest snow was in the deciduous-dominated forest, with mixed and coniferous forests having similar snow depths. The mean difference between the magnaprobe and snow tube snow depths is a modest 1.3 cm in the field and 1.9 cm in the forest, with differences of 1.9, 2.0, and 1.9 cm in the deciduous, mixed, and coniferous land types, respectively. However, the differences between the magnaprobe and snow tube snow depths in the forest were higher on 18 December (2.5 cm) than on 4 February and 24 February (1.7, and 1.4 cm, respectively). Based on t-test results, the magnaprobe measured significantly deeper snow depth compared to the snow tube in both the field and the forest regardless of whether individual locations (p value <0.001) or grid cell average snow depths (p value = 0.02) were used. Based on Welch's adjusted ANOVA test, there are no significant differences in overprobing among forest land types (p value = 0.24). The RMSD values between the magnaprobe and snow tube snow depths are 3.0 cm (2.3 cm) and 2.5 cm (2.0 cm) for the forest and field sampling sites (grid average values), respectively. Thus, the sampling method has a different impact in the field than in the forest, and the RMSD and bias values provide an indicator of the different errors associated with in situ measurements based on land type when used for model or remote sensing validation. While these differences are significant, the average litter depths exceeded the differences between the magnaprobe and snow tube snow depths in the forest, which were 2.5, 1.7, and 1.4 cm on 18 December, 4 February, and 24 February, respectively.
This study quantifies the differences between snow depth measurements made with a magnaprobe and with a snow tube. The differences seem to be primarily associated with greater overprobing by the magnaprobe into vegetation or organic layers and thawed soils, though in some cases, the large differences could instead be due to the larger-diameter snow tube hitting a branch from a down tree or debris that the magnaprobe bypassed. The result was that the magnaprobe snow depth measurements were higher than snow tube measurements, with a greater difference in the forest than in the field. An average of 5 cm bias occurred in the tundra matte during the Cold Land Processes Experiment (CLPX) Alaska campaign (Sturm and Holmgren, 2018). Also, in the open-tundra environment, a 7.6 cm average overprobing penetration for approximately 40 cm deep snow was found (Toose et al., 2020). Berezovskaya and Kane (2007) also noted overprobing of 5 to 9 cm with a magnaprobe as compared to a snow tube and found a bias in northern Alaska for snow depths between 29 and 48 cm. In this study, the overprobing (1.3 cm in the field and a 1.9 cm in the forest) was less than in previous studies, probably due to the lower range of snow depth and different surface conditions as compared to previous studies.
We also agree with López-Moreno et al. (2020) about the fact that it is important to understand the snowpack and land conditions for which an individual sampler was designed to select the most appropriate sampler. Understanding leaf litter or vegetation depths and underlying soils may potentially reduce and help to account for the overprobing errors of magnaprobe snow depth measurements. Sturm and Holmgren (2018) suggested that operators need to learn to push a magnaprobe through snow but not penetrate too deeply the underlying vegetation or organic layers by developing a sense for the base of the snowpack. This recommendation may be difficult to implement (e.g., over soft vegetation) where the probe easily penetrates the vegetation and may be problematic if multiple operators apply different amounts of force (Berezovskaya and Kane, 2007). If operators overprobe the base of the (frozen) soils, one option is to consistently measure the depths in the same way (which would be snow depth plus vegetation) and then subtract typical vegetation depths in the study area from the depths. When leaf litter is evident, penetration of the organic layer should be quantified by using independent leaf litter measurements, preferably using the snow depth sampling instrument, to bias-correct snow depths.
As observed in this study, leaf litter and soil frost may differentially impact in situ snow depth sampling methods. The earliest sampling campaign had limited soil frost and likely reduced litter compaction. Distinct contributions of forest leaf litter depth to magnaprobe and snow tube snow depths may occur because the narrow magnaprobe fully penetrates the leaf litter while the larger-diameter snow tube only partially penetrates the litter, or the magnaprobe may only partially penetrate the leaf litter while the snow tube does not break through the leaf litter. Partial penetration by the magnaprobe into the leaf litter layer (i.e., overprobing) may vary by the freeze–thaw state of the duff layer and/or mineral soil layers beneath the leaf litter layer. The horizontally aligned, matted leaf litter could also limit snow tube penetration. High spatial variability of leaf litter depth could also be a factor, though this was not quantified here. Thus, the increased differences among in situ methods in forested areas observed in this study point to the particular importance of in situ validation in forested areas and, more generally, sampling with multiple methods in an area with a non-uniform underlying substrate.
In summary, there are three major suggestions from this work, which are outlined below.
-
With an ephemeral snowpack in forested environments, snow depth measurements using a Federal snow tube likely avoid overprobing, which can frequently occur when a magnaprobe is used.
-
The use of the average of multiple-point samples within a grid is recommended instead of single measurements because the average of multiple-point samples can reduce the point-to-point variability and spatial representativeness errors.
-
Measurements of vegetation, leaf litter, and soil frost can help to account for the errors of in situ snow depth observations, particularly when using a magnaprobe.
Manual, in situ sampling snow depth measurements can be made quickly and easily, but making consistent, representative, and unbiased measurements can be challenging when the surface is irregular, when the vegetation or organic layers and unfrozen soils result in overprobing, and when the leaf litter compacts during the winter. This study quantified the differences between snow depth measurements made with a magnaprobe and a Federal snow tube in a mixed-use temperate forest landscape with ephemeral snowpack. For all sampling campaigns and land cover types, the magnaprobe snow depth measurements (mean 14.9 cm) were usually, but not always, deeper than the snow tube measurements (13.2 cm) and had a 1.7 cm average difference. For these shallow snowpacks, this amounts to a 12 % difference, but in a deeper snowpack, the relative impact of this difference would be much smaller. Biases were significantly higher in the forest (1.9 cm) than the field (1.3 cm). The difference between the two instruments was 50 % higher in the early-winter campaign than in the later campaigns. The differences among measurement techniques in this present study reflect the current study area, surface conditions for a single season, and the operation of the instruments by this project team. Further studies to understand the errors from in situ sampling using snow probes are warranted in various snow environments with different vegetation and soil conditions to provide guidance on the best practices for using in situ snow probe datasets under conditions where overprobing is likely.
The in situ snow observations are available in the Supplement.
The supplement related to this article is available online at: https://doi.org/10.5194/tc-17-3435-2023-supplement.
HP, JMJ, EAB, AGH, FBS, MP, and EC designed the research. HP, CW, JMJ, AGH, FBS, MP, EAB, and EC conducted fieldwork to obtain lidar and/or in situ snow observations. HP, CW, JMJ, EAB, AGH, and MP performed the analysis. HP, EC, and AGH produced the figures. HP, JMJ, EAB, and EC wrote the initial draft. All authors contributed to the review and editing of the paper.
The contact author has declared that none of the authors has any competing interests.
Publisher’s note: Copernicus Publications remains neutral with regard to jurisdictional claims in published maps and institutional affiliations.
Approved for Public Release. Distribution is Unlimited. This material is based upon work supported by the Broad Agency Announcement Program and the Cold Regions Research and Engineering Laboratory (ERDC-CRREL) under contract nos. W913E518C0005 and W913E521C0006. The authors are grateful to Lee Friess for providing a technical review of the draft paper, Mahsa Moradi Khaneghahi for supporting the paper preparation, and Brigid Ferris for training the team on litter depth sampling. We thank Christina Herrick for post-processing the GPS data.
This research has been supported by the U.S. Department of Defense (grant nos. W913E521C0006 and W913E518C0005).
This paper was edited by Jürg Schweizer and reviewed by A. N. Arslan and Ryan Webb.
Berezovskaya, S. and D. L. Kane: Measuring snow water equivalent for hydrological applications: part 1, accuracy of observations, in: Proceedings of the 16th International Northern Research Basins Symposium and Workshop, 27 August–2 September, 2007, Petrozavodsk, Russia, 29–37, 2007.
Bongio, M., Arslan, A. N., Tanis, C. M., and De Michele, C.: Snow depth time series retrieval by time-lapse photography: Finnish and Italian case studies, The Cryosphere, 15, 369–387, https://doi.org/10.5194/tc-15-369-2021, 2021.
Clyde, G. D.: Circular No. 99 – Utah Snow Sampler and Scales for Measuring Water Content of Snow, UAES Circulars, Paper 90, https://digitalcommons.usu.edu/uaes_circulars/90 (last access: 22 August 2023), 1932.
Derry, J., Kane, D., Lilly, M., and Toniolo, H.: Snow-course measurement methods, North Slope, Alaska, University of Alaska Fairbanks, Water and Environmental Research Center, Report INE/WERC, 15, http://www.arctic-transportation.org/doc/ADOT_NS_RPT0807_Final.pdf (last access: 22 August 2023), 2009.
Dixon, D. and Boon, S.: Comparison of the SnowHydro snow sampler with existing snow tube designs, Hydrol. Process., 26, 2555–2562, 2012.
Elder, K., Rosenthal, W., and Davis, R. E.: Estimating the spatial distribution of snow water equivalence in a montane watershed, Hydrol. Process., 12, 1793–1808, 1998.
Farnes, P. E., Goodison, B. E., Peterson, N. R., and Richards, R. P.: Metrication of manual snow sampling equipment, Final report Western Snow Conference, 19–21, https://westernsnowconference.org/sites/westernsnowconference.org/PDFs/1982Farnes.pdf (last access: 22 August 2023), 1983.
Gandahl, R.: Determination of the depth of soil freezing with a new frost meter, Rapport, 20, 3–15, 1957 (in Swedish).
Goodison, B., Glynn, J., Harvey, K., and Slater, J.: Snow surveying in Canada: A perspective, Can. Water Resour. J., 12, 27–42, 1987.
Kaspari, M. and Yanoviak, S. P.: Biogeography of litter depth in tropical forests: evaluating the phosphorus growth rate hypothesis, Funct. Ecol., 22, 919–923, 2008.
Kinar, N. and Pomeroy, J.: Measurement of the physical properties of the snowpack, Rev. Geophys., 53, 481–544, 2015.
Kopp, M., Tuo, Y., and Disse, M.: Fully automated snow depth measurements from time-lapse images applying a convolutional neural network, Sci. Total Environ., 697, 134213, https://doi.org/10.1016/j.scitotenv.2019.134213, 2019.
Leppänen, L., Kontu, A., Hannula, H.-R., Sjöblom, H., and Pulliainen, J.: Sodankylä manual snow survey program, Geosci. Instrum. Meth., 5, 163–179, 2016.
López-Moreno, J. I., Fassnacht, S. R., Heath, J. T., Musselman, K. N., Revuelto, J., Latron, J., Morán-Tejeda, E., and Jonas, T.: Small scale spatial variability of snow density and depth over complex alpine terrain: Implications for estimating snow water equivalent, Adv. Water Resour., 55, 40–52, https://doi.org/10.1016/j.advwatres.2012.08.010, 2013.
López-Moreno, J. I., Leppänen, L., Luks, B., Holko, L., Picard, G., Sanmiguel-Vallelado, A., Alonso-González, E., Finger, D. C., and Arslan, A. N.: Intercomparison of measurements of bulk snow density and water equivalent of snow cover with snow core samplers: Instrumental bias and variability induced by observers, Hydrol. Process., 34, 3120–3133, https://doi.org/10.1002/hyp.13785, 2020.
Perron, C. J., Bennett, K., and Lee, T. D.: Forest stewardship plan: Thompson farm, NH, University of New Hampshire, Ossipee Mountain Land Company, West Ossipee, https://universitysystemnh.sharepoint.com/teams/COLSASocialMedia/Shared Documents/Forms/AllItems.aspx?id=%2Fteams%2FCOLSASocialMedia%2FShared Documents%2FWebsite%2Fthompson%2Dfarm%2Dplan%2Epdf&parent=%2Fteams%2FCOLSASocialMedia%2FShared%20Documents%2FWebsite&p=true&ga=1 (last access: 16 August 2023), 2004.
Pirazzini, R., Leppänen, L., Picard, G., Lopez-Moreno, J. I., Marty, C., Macelloni, G., Kontu, A., Von Lerber, A., Tanis, C. M., Schneebeli, M., and De Rosnay, P.: European in-situ snow measurements: practices and purposes, Sensors, 18, 1–51, https://doi.org/10.3390/s18072016, 2018.
Raleigh, M. S. and Small, E. E.: Snowpack density modeling is the primary source of uncertainty when mapping basin-wide SWE with lidar, Geophys. Res. Lett., 44, 3700–3709, 2017.
Sturm, M. and Holmgren, J.: An automatic snow depth probe for field validation campaigns, Water Resour. Res., 54, 9695–9701, 2018.
Sturm, M., Taras, B., Liston, G. E., Derksen, C., Jonas, T., and Lea, J.: Estimating snow water equivalent using snow depth data and climate classes, J. Hydrometeorol., 11, 1380–1394, 2010.
Toose, P., King, J., Silis, A., and Derksen, C.: TVCSnow 2017–2018 tundra snow depth probe measurements (Version 1), Zenodo [data set], https://doi.org/10.5281/zenodo.4021328, 2020.
Walker, B., Wilcox, E. J., and Marsh, P.: Accuracy assessment of late winter snow depth mapping for tundra environments using Structure-from-Motion photogrammetry, Arctic Sci., 7, 588–604, https://doi.org/10.1139/as-2020-0006, 2020.