the Creative Commons Attribution 4.0 License.
the Creative Commons Attribution 4.0 License.
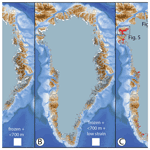
Drill-site selection for cosmogenic-nuclide exposure dating of the bed of the Greenland Ice Sheet
Jason P. Briner
Caleb K. Walcott
Joerg M. Schaefer
Nicolás E. Young
Joseph A. MacGregor
Kristin Poinar
Benjamin A. Keisling
Sridhar Anandakrishnan
Mary R. Albert
Tanner Kuhl
Grant Boeckmann
Direct observations of the size of the Greenland Ice Sheet during Quaternary interglaciations are sparse yet valuable for testing numerical models of ice-sheet history and sea level contribution. Recent measurements of cosmogenic nuclides in bedrock from beneath the Greenland Ice Sheet collected during past deep-drilling campaigns reveal that the ice sheet was significantly smaller, and perhaps largely absent, sometime during the past 1.1 million years. These discoveries from decades-old basal samples motivate new, targeted sampling for cosmogenic-nuclide analysis beneath the ice sheet. Current drills available for retrieving bed material from the US Ice Drilling Program require < 700 m ice thickness and a frozen bed, while quartz-bearing bedrock lithologies are required for measuring a large suite of cosmogenic nuclides. We find that these and other requirements yield only ∼ 3.4 % of the Greenland Ice Sheet bed as a suitable drilling target using presently available technology. Additional factors related to scientific questions of interest are the following: which areas of the present ice sheet are the most sensitive to warming, where would a retreating ice sheet expose bare ground rather than leave a remnant ice cap, and which areas are most likely to remain frozen bedded throughout glacial cycles and thus best preserve cosmogenic nuclides? Here we identify locations beneath the Greenland Ice Sheet that are best suited for potential future drilling and analysis. These include sites bordering Inglefield Land in northwestern Greenland, near Victoria Fjord and Mylius-Erichsen Land in northern Greenland, and inland from the alpine topography along the ice margin in eastern and northeastern Greenland. Results from cosmogenic-nuclide analysis in new sub-ice bedrock cores from these areas would help to constrain dimensions of the Greenland Ice Sheet in the past.
- Article
(14131 KB) - Full-text XML
-
Supplement
(25693 KB) - BibTeX
- EndNote
Recent observations reveal significant ice loss in Greenland and Antarctica, with the Greenland Ice Sheet (GrIS) presently contributing more to sea level rise than the Antarctic Ice Sheet (AIS) (The IMBIE team, 2018, 2020). The higher potential for portions of the AIS to collapse due to marine ice-sheet instability, however, leaves estimates of future sea level rise highly uncertain (Scambos et al., 2017; DeConto et al., 2021; Edwards et al., 2021). Non-linearities in ice-sheet response to climate change also apply to the GrIS, which has been simulated to disappear in as little as one millennium (Aschwanden et al., 2019). Estimated rates of GrIS loss this century under the current trajectory of greenhouse-gas emissions (Goelzer et al., 2020; Edwards et al., 2021) have been shown to exceed those under natural variability over the past 12 000 years (Briner et al., 2020).
Although present rates of ice-sheet loss are exceptional and concerning, there are few direct constraints on GrIS and AIS response to similar warmth during past interglaciations of the Quaternary (e.g., de Vernal and Hillaire-Marcel, 2008; Schaefer et al., 2016). Thus, knowledge of ice-sheet response under past climates that are comparable to the climate of our near future remains limited. Proxy data from geological archives, such as sedimentological characteristics in adjacent seas, have been used to evaluate ice-sheet history, although these provide indirect evidence only for past changes in ice-sheet size. A growing body of evidence from offshore Greenland documents overall ice-sheet growth and its subsequent oscillatory configurations throughout the Pliocene and Quaternary (e.g., Bierman et al., 2016; Knutz et al., 2019). Paleoceanographic studies have made valuable inferences of climate conditions (e.g., de Vernal and Hillaire-Marcel, 2008; Cluett and Thomas, 2021) and ice-sheet configuration (e.g., Reyes et al., 2014; Hatfield et al., 2016) during brief interglacials. Generating direct knowledge of past GrIS response to interglacial warmth has proven difficult with these approaches. Far-field sea level reconstructions help to constrain GrIS response during past interglaciations (e.g., Dyer et al., 2021) yet still benefit from direct observations from individual ice sheets. Ice-sheet modeling has simulated a variety of ice-sheet volumes and configurations during past interglaciations (e.g., Goelzer et al., 2016; Robinson et al., 2017; Plach et al., 2018; Sommers et al., 2021), indicating more geologic measurements of ice-sheet extent are needed to evaluate these results.
The age of ice in basal ice core sections has been used to constrain the GrIS configuration during marine isotope stage (MIS) 5e (129–116 ka) and thus validate numerical simulations of ice size and configuration during the last interglacial (Otto-Bleisner et al., 2006; Plach et al., 2018; Domingo et al., 2020). However, there is some uncertainty about the role that ice advection plays in bringing aged ice over a previously ice-free location. For example, Yau et al. (2016a) found that the best fitting models for matching their elevation and temperature reconstructions for NEEM and GISP2 did not have ice at NEEM during MIS 5e, implying that the MIS 5e ice recovered at NEEM today not only flowed laterally but re-advanced over a deglaciated landscape. This phenomenon can be observed directly at the modern ice-sheet margin today, where Pleistocene-age ice outcrops at the margin in western Greenland (e.g., Reeh et al., 2002; MacGregor et al., 2020) where there was no ice as recently as the middle Holocene (Briner et al., 2010). Thus, it is critical to obtain independent information about sub-ice bedrock exposure age because apparently the age/stratigraphy of the overlying ice does not necessarily provide a continuous constraint on ice-cover history.
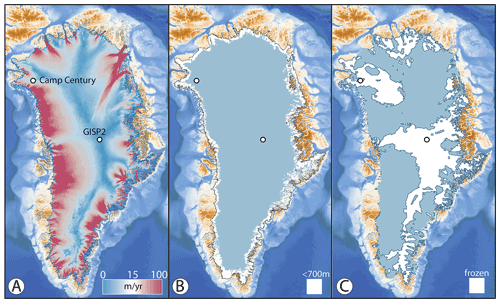
Figure 1(a) Horizontal surface velocities of the Greenland Ice Sheet; the slowest flowing areas define the summit ridge and ice divides; velocity from Greenland Ice Sheet velocity map from Sentinel-1, winter campaign 2019/2020 (version 1.3); QGreenland v2.0. (b) The pattern of < 700 m ice thickness (white) shown around perimeter of the ice sheet, which covers 15.2 % of the ice-sheet footprint. (c) Where the basal thermal state is likely frozen bedded (white), which covers 37.4 % of the ice-sheet footprint (from MacGregor et al., 2022). Basemap topography and bathymetry from Morlighem et al. (2017).
Fortunately, a new frontier of science is emerging, aimed at generating direct constraints on former ice-sheet size using information collected from the ice-sheet bed. Schaefer et al. (2016) measured cosmogenic 10Be and 26Al in bedrock obtained below the GISP2 ice core (Fig. 1), equipped with updated procedures and vastly improved analytic sensitivity relative to an earlier attempt (Nishiizumi et al., 1996). Their measurements require the GrIS to have been absent at the GISP2 locality for 280 kyr of the past 1.4 Myr. Although alternative histories are possible, the results lead to an important conclusion: an almost entirely absent ice sheet in Greenland w0ithin the last 1.1 Myr. Furthermore, these types of data directly constrain past ice-sheet configurations, unlike marine sediment records from adjacent seas that provide indirect evidence. More recently, Christ et al. (2021) measured cosmogenic 10Be and 26Al in re-discovered sub-ice sediments in the Camp Century ice core collected in the 1960s (Fig. 1). They interpret their results to indicate that the landscape below Camp Century became ice-free at least once in the last 1.0 Myr. While one might expect the GrIS flank site of Camp Century to become ice-free during some interglacial periods (model simulations commonly show this; Plach et al., 2018; Sommers et al., 2021), the findings from beneath the summit of the GrIS were more unexpected because model simulations rarely show ice-free conditions there. Additionally, new approaches have been developed to solve for long-term ice-sheet occupation and subglacial erosion histories from vertical profiles of cosmogenic nuclides measured in multiple meters of rock core (Balter-Kennedy et al., 2021). Performing such analyses on new multi-meter-long bedrock cores from beneath the GrIS will be key for deciphering GrIS history.
Cosmogenic-nuclide measurements from sub-ice bed material in Greenland already have been shown to place direct constraints on past ice-sheet history, despite the study of only two cosmogenic isotopes (10Be and 26Al) in these samples thus far. Additionally, the recent results from the sub-GrIS environment, although derived using legacy material from sites not targeted for cosmogenic-nuclide measurements, have demonstrated the power of this approach. While drilling technology that allows quick access (i.e., in a single field season) to the bed below ice-sheet summits is being developed for application in Antarctica (Goodge and Severinghaus, 2016; Goodge et al., 2021), there is no such drill – or plans for one – to operate in Greenland. However, there are drills designed to quickly access the bed in locations where ice thickness is < 700 m (Spector et al., 2017, 2018). The goal of this study is to survey Greenland to identify sites that are potentially suitable for sub-ice cosmogenic-nuclide measurements using two suitable drills in the US Ice Drilling Program's inventory: the Agile Sub-Ice Geological (ASIG; Kuhl et al., 2021) Drill and the Winkie Drill (Boeckmann et al., 2021). Both of these drills can operate in Greenland. Considering drill specifications and scientific and safety criteria, we identify multiple suitable sites near the GrIS margin across northern and eastern Greenland. These sites represent candidate targets for the GreenDrill project supported by the US National Science Foundation.
The drills currently available from the US Ice Drilling Program that are designed to drill rock cores beneath tens to hundreds of meters of glacial ice require the bed beneath the ice to be frozen to its bed. Additional specifications for scientific projects focused on sub-ice samples obtained via drilling, such as bedrock lithology and site accessibility, further limit suitable areas. The bedrock lithology of Greenland is varied and is only exposed around the island's perimeter and directly observable in only one hand sample from the base of the GISP2 ice core site. With only six locations across the GrIS interior where boreholes have reached the bed, there are also limited direct observations of the ice sheet's basal thermal state. Below, we compile this and other necessary information for identifying potential sites for retrieval of rock cores beneath the GrIS.
2.1 Drills
We first briefly outline the technical requirements of the two presently available US Ice Drilling Program drills designed to drill through ice and into the underlying bedrock: ASIG Drill and Winkie Drill (Albert et al., 2021). The ASIG Drill is currently designed to drill access holes through ice < 700 m thick and collect bedrock cores several meters long. It requires frozen basal conditions to ensure that drilling fluid is maintained in the entire borehole across the ice–bed interface. The ASIG Drill was successfully used in West Antarctica near the Pirrit Hills in 2016–2017, where it drilled through approximately 150 m of ice and collected 8 m of 39 mm diameter rock core of excellent quality (Kuhl et al., 2021). Nearly 5 m of ice core was also collected near the ice–bedrock transition; however, the core quality was poor. The Winkie Drill is capable of drilling 120 m of ice and rock (e.g., it can retrieve a 10 m rock core from beneath 110 m of ice); it also has the requirement of a frozen bed. Given these restrictions, the Winkie Drill is mostly restricted to frozen-bedded environments very near the GrIS margin, and the ASIG Drill is suitable to drill in similar environments slightly farther inland.
2.2 Ice thickness
The large-scale thickness of the GrIS is relatively well known, stemming from several decades of radar data collection by NASA and European institutions (e.g., Li et al., 2012). Morlighem et al. (2017) combined airborne radar-sounding-derived ice thickness data with comprehensive, high-resolution ice motion measurements derived from satellite interferometric synthetic-aperture radar. This combination of datasets allowed Morlighem et al. (2017) to employ a mass conservation algorithm (Morlighem et al., 2011; McNabb et al., 2012) to calculate ice thickness around the periphery of the GrIS. They produced a map of bed topography by subtracting ice thickness from a digital elevation model of the ice surface. Mass conservation works best in areas of fast flow, where uncertainty in flow direction is small and the glaciers mostly flow due to basal motion (Morlighem et al., 2011). In the interior, where deformation is likely a more dominant component of ice flow and uncertainty in flow direction is greater, they employed ordinary kriging to interpolate ice thickness measurements. We use BedMachine v3 (Morlighem et al., 2017) and ArcGIS to deduce that 15.2 % of the GrIS is < 700 m in thickness (Fig. 1b).
2.3 The basal thermal state of the GrIS
Due to the limited number of boreholes that have reached the GrIS bed, its basal thermal state must presently be estimated from a synthesis of multiple methods. MacGregor et al. (2016, 2022) combined thermomechanical ice-flow models and inferences from airborne and satellite remote sensing to constrain where the bed is likely thawed, where it is likely frozen and where it remains too uncertain to specify, at a spatial resolution of 5 km. The latest version of this synthesis of the GrIS likely basal thermal state (MacGregor et al., 2022) is shown in Fig. 1c. The map suggests frozen-bedded conditions across 37.4 % of the ice sheet, mostly beneath ice divides and parts of North Greenland (Fig. 2). The ice margin and near-ice-margin areas throughout most of Greenland are largely believed to be thawed, except for a few locations across North and East Greenland where frozen-bedded conditions are ubiquitous – even near the ice margin. However, there are many areas where the basal thermal state is mapped as uncertain (i.e., areas that are inconclusive in terms of their likelihood to be either warm bedded or frozen bedded), and many of these areas also extend to the ice margin in portions of North and East Greenland. Jordan et al. (2018) used radar returns to identify locations of probable water at the bed. Although the method could not be applied throughout Greenland due to limitations in radar extent and quality, their fine-resolution dataset was included by MacGregor et al. (2022). Bedrock weathering textures and landforms observed in landscapes occupied by Pleistocene ice sheets reveal sharp transitions between warm- and frozen-bedded conditions in the past, particularly in areas of high topographic relief (e.g., Sugden, 1978; Briner et al., 2006). Thus, there could be localized patches of frozen-bedded conditions across many areas around the GrIS perimeter that are too small in scale to be suitably represented using the methods of Jordan et al. (2018) and MacGregor et al. (2022). Combining likely frozen basal conditions with ice thicknesses < 700 m results in 6.8 % of the bed available for drilling (Figs. 2 and 3a).
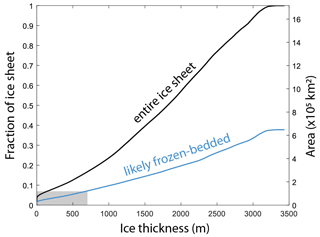
Figure 2Greenland Ice Sheet thickness versus area. Plot shows that only about one-third of the ice sheet by area is likely frozen-bedded and thus available for subglacial access. The current limit of drills operating under 700 m ice thickness further reduces available portion of the ice-sheet bed for access (gray area). Note that increasing a drill's depth capability increases the area of the bed available for drilling; the ability to drill into wet-based sections of the ice sheet would significantly increase the area available for drilling.
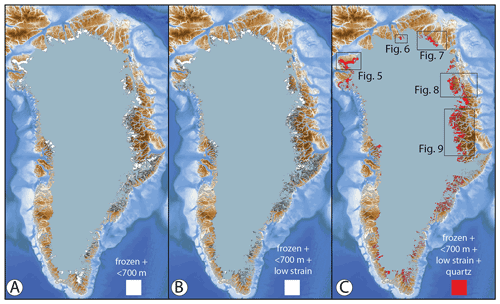
Figure 3(a) Portion of the Greenland Ice Sheet bed (6.8 %) that is both likely frozen and beneath < 700 m of ice. (b) Same as in panel (a) but with a low likelihood of surface crevasses (4.8 %). (c) Same as in panel (b) but with likely quartz-bearing lithologies (3.4 %). Basemap topography and bathymetry from Morlighem et al. (2017).
Finally, prior to drilling, the selected sites should be assessed with geophysical methods to further estimate the thermal state of the bed. Existing radar profiles combined with new radar and seismic measurements can reduce the uncertainty about the condition of the bed. Seismic methods can more confidently measure whether a significant water volume is present at the bed, either pooled or within sediment pores (e.g., Kulessa et al., 2017). The reflectivity of water or water-laden sediments is significantly different than for frozen sediments. Note that a thin layer of water over crystalline bedrock would be difficult to distinguish from frozen ice over bedrock.
2.4 Surface features, safety and site accessibility
Because available drills require < 700 m ice thickness, the viable areas of interest are mostly restricted to near the ice margin (Fig. 1b). These areas generally have high surface velocity (> 50 m yr−1) and spatial variability in surface velocity as ice flow becomes increasingly influenced by underlying topography (Fig. 1a). Consequently, these areas have high strain rates and can be heavily crevassed, making them some of the most dangerous locations on the GrIS to work. However, not all ice-marginal areas exhibit high velocity and high strain rates, so some areas are relatively crevasse-free. Surface strain rates derived from GrIS surface velocity (Fig. 1a) can guide site selection for low likelihood of crevassing. In this way, one can address the criterion of being most likely to be safe for air support and/or access via traverse vehicles. We use a strain-rate field from Poinar and Andrews (2021) and a threshold value of 0.005 per year, above which crevasses are likely to form (Joughin et al., 2013). This analysis further reduces the area of the GrIS suitable for drilling from 6.8 % to 4.8 % (Fig. 3b).
2.5 Cosmogenic nuclides and subglacial geology
An entire family of cosmogenic nuclides is routinely measured in Earth materials. Most research to date in Earth science, however, has used cosmogenic nuclides produced in quartz: 26Al, 14C and 10Be (Granger et al., 2013; Briner et al., 2014; Balco, 2020). While there are cosmogenic nuclides that can be used in mafic lithologies (e.g., 36Cl and 3He) and carbonates (e.g., 36Cl), the advantage of quartz is that the trio of 26Al, 14C and 10Be can all be measured together (e.g., Young et al., 2021). These three nuclides have widely spaced half-lives, providing a powerful exposure–burial chronometer well suited for providing direct constraints on ice-sheet history. Additionally, 36Cl can also be measured in feldspars, and thus targeting felsic-crystalline lithologies potentially offers a fourth cosmogenic nuclide with a unique half-life for analysis.
Because 81 % of Greenland's land area lies beneath the ice, bedrock geology has only been mapped across 19 % of Greenland. There is a large degree of uncertainty about the lithology below the ice sheet. Dawes (2009) inferred the sub-GrIS geology based on information from six methods: drill sites, nunataks, coast-to-coast correlation, glacial erratics, detrital provenance studies and geophysics. For the purpose of identifying sites for cosmogenic-nuclide analysis, we provide a highly abbreviated overview of this geology with particular attention paid to quartz-bearing lithologies in areas likely to coincide with frozen-bedded conditions. We use the geologic map of Greenland, available online at https://www.greenmin.gl/ (last access: July 2022; Pedersen et al., 2013; Henriksen et al., 2009). Generally, Greenland mostly consists of Precambrian shield rocks (both Archean and Proterozoic; largely quartz bearing) in its southern, western and central areas. North Greenland consists of Paleozoic basins containing mostly non-quartz-bearing lithologies. East and Northeast Greenland comprise the Caledonian fold belt and a complex pattern of Proterozoic rocks of mixed lithology, although these are thought to be mainly limited to the island's periphery. Portions of the central east and central west coasts of Greenland contain Paleogene volcanic lithologies that may connect beneath the central GrIS. North Greenland generally encompasses the highest proportion of the margin and near-margin areas thought to be frozen bedded; however, carbonate and other non-quartz-bearing lithologies dominate these areas. We use the geologic map of Greenland to categorize bedrock lithology into quartz-bearing and non-quartz-bearing units (Fig. 4). We remove the ice-marginal areas adjacent to carbonate and volcanic lithologies from consideration, which reduces the target area from 4.8 % to 3.4 % of the GrIS (Fig. 3c; see Supplement for geographic-information-system data layers).
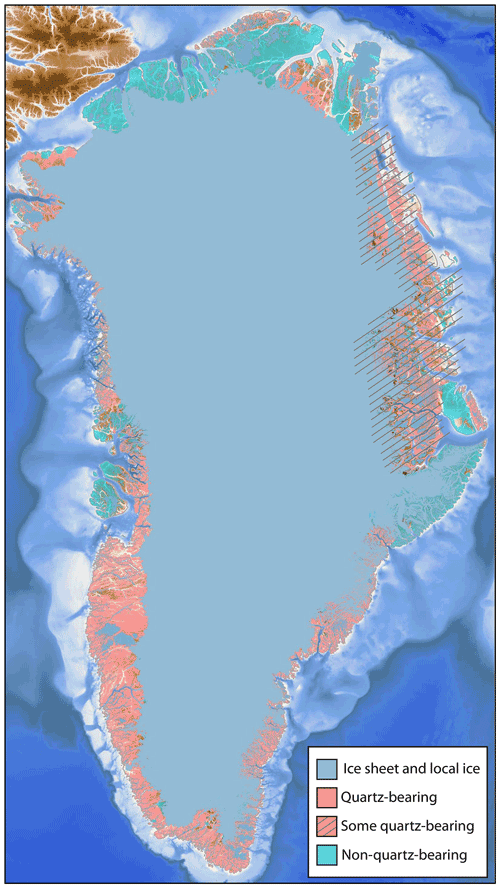
Figure 4Simplified bedrock geology map of Greenland, showing lithology sub-divided into probably quartz-bearing rocks, some quartz-bearing lithologies and probably non-quartz-bearing rocks. From https://www.greenmin.gl/. Basemap topography and bathymetry from Morlighem et al. (2017).
Cosmogenic-nuclide analyses made in a depth profile below the ice–bed interface yield important information. Measurements in a rock core spanning 1 m or more, for example, can allow one to easily identify whether or not the current ice–bed interface has been eroded and/or covered by snow, ice or sediment for long durations (e.g., Schaefer et al., 2016). On the other hand, one cannot determine with surface-only samples whether a surface has been impacted by minor erosion and/or burial by snow, ice or sediment. Thus, analysis of bedrock cores is most important for elucidating ice-sheet histories from cosmogenic-nuclide inventories. Furthermore, cores spanning several meters and including depths dominated by muon production have the added advantage of constraining orbital-scale term exhumation histories (e.g., Balter-Kennedy et al., 2021).
Sampling from a bedrock substrate has advantages over samples from sediment deposits, although cosmogenic-nuclide measurements from both are informative. Sediments beneath ice sheets are more easily eroded, deformed, entrained, transported and re-deposited than bedrock. Thus, cosmogenic-nuclide concentrations from the sediment grains themselves, which have a transport and deposition history, are more complicated to interpret than those in bedrock. Furthermore, cold-based ice that flows atop sediment sections can more easily erode a sediment surface (via entrainment processes) than in bedrock substrates. Thus, not only is a cosmogenic signal in sediments derived from each individual grain's exposure and burial elsewhere (which are later amalgamated into a single deposit), but the ice bed itself may not represent a prior land surface. Thus, sediment samples could be from an arbitrary depth below a paleo-surface. The depositional environment of sediment is also important. If ice overlies a fluvial sediment sequence, then the cosmogenic-nuclide inventory is highly likely to have a complicated genesis and thus a more complicated interpretation. On the other hand, if the sediment is saprolite or regolith, and largely formed in situ, then its cosmogenic-nuclide inventory likely would be more straightforward to interpret. In any case, for targeted sub-GrIS cosmogenic-nuclide campaigns, the highest priority sites are those where non-erosive ice rests directly on quartz-bearing bedrock.
How to maximize the chance of drilling into bedrock? Site selection is aided by airborne radar sounding data obtained by NASA Operation IceBridge. Existing surveys of the ice-sheet bed are inadequate for identifying every low topographic swale that could potentially be sediment filled, particularly between radar flight lines. However, by avoiding valleys and low areas and instead opting for mountain summits or plateaus, we can increase the likelihood of drilling into bedrock with thin or no sediment cover. Although not always the case, in most areas of Greenland that are ice-free today, bare bedrock surfaces generally exist in higher proportion on hilltops and uplands, as opposed to low-lying areas and valley bottoms. Thus, choosing sites along radar flight lines ensures the most reliable knowledge of bed topography and ice thickness at a candidate drill site.
2.6 Strategizing drill-site selection related to scientific motivation
Having applied above the drilling and geologic requirements for site selection, we next consider the scientific progress that could be realized from the analysis of bed materials at a particular site. With the goal of constraining Pleistocene GrIS history in mind, we consider four primary criteria.
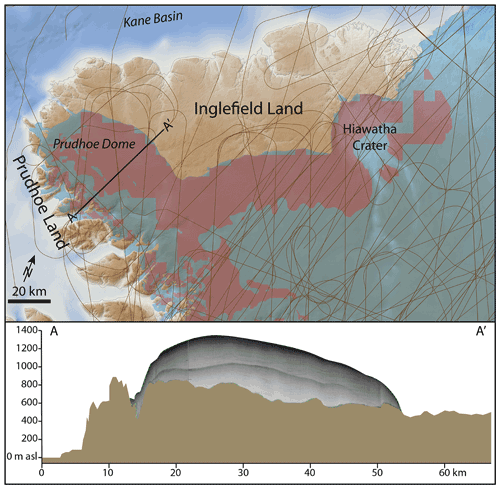
Figure 5Top panel shows areas that meet drilling requirements (shown in red) in NW Greenland. The Greenland Ice Sheet is depicted in light blue, and NASA Operation IceBridge (OIB) flight lines are shown as thin brown lines. Bottom panel shows OIB radar of Prudhoe Dome along A–A′, with topography, the ice-sheet bed and the ice-sheet surface from radar collected in 2017; the mid-ice-sheet reflector is the surface multiple. Basemap topography and bathymetry from Morlighem et al. (2017).
First, the best sites should be robust monitors of past ice-sheet margin change. There could be regions, such as high-elevation terrain (e.g., in mountainous East Greenland), that meet the technical criteria but retain local ice cover during times of reduced ice-sheet configurations, complicating the link between the study site and broader GrIS change. There could also be sites that are part of the GrIS but are better conceived of as separate ice domes connected to the ice sheet via a saddle; these local domes may persist longer than the adjacent ice sheet during interglacial periods as disconnected ice caps (e.g., Prudhoe Dome, Fig. 5). Ice-sheet modeling should be used to help guide site selection (Keisling et al., 2022).
Second, to best capitalize on new measurements of cosmogenic-nuclide signatures of past ice-sheet changes (Spector et al., 2018; Keisling et al., 2022), sites that have persistent frozen-bedded conditions throughout glacial–interglacial cycles should be sought. These sites should favor preservation of cosmogenic nuclides at the ice–bed surface and reduce the likelihood of significant periods of time with subglacial erosion that removes the cosmogenic-nuclide inventory. At sites where sub-glacial conditions favor erosion, cosmogenic nuclides would be largely absent, hence removing one of the main reasons for obtaining sub-ice bedrock samples. Identifying these sites could be based on a combination of selecting presently-frozen-bedded areas, favoring high-elevation locations or ice divide areas likely to be frozen bedded during past larger ice-sheet configurations, and evaluating paleo-ice-sheet models to find ideal drilling locations.
Third, there may be some sites that are more sensitive monitors of reduced ice extent than others. For example, while some sites at 600 m ice thickness today may become ice-free at under 5 % reduction in ice-sheet mass, others may not become ice-free until a substantially greater reduction in mass. Using numerical ice-sheet models could greatly assist site selection and help to further explore sites that meet the technical requirements for their potential to constrain past ice-sheet configurations (Keisling et al., 2022).
Fourth, some ice-sheet margin areas that include large, fast-flowing outlet glaciers with beds below sea level (e.g., near Jakobshavn Isbræ, Petermann Glacier, Northeast Greenland Ice Stream) could potentially collapse at rates faster than other ice-sheet margin areas. Thus, sites neighboring these regions, such as the Northeast Greenland Ice Stream, could not only serve as a binary signal of ice-sheet presence/absence but could help to elucidate the response of major outlet glaciers influenced by ice–ocean interactions to past climate forcing. Does the Northeast Greenland Ice Stream collapse during past warm times and exhibit proportionally more ice-sheet recession than other ice-sheet sectors? Sites adjacent to the Northeast Greenland Ice Stream could help resolve this question.
Finally, additional considerations relating to field season planning could lead to meeting scientific goals most efficiently. Multiple drill cores along transects (even including sites beyond the present – ephemeral – position of the ice-sheet margin) could boost confidence in constraining past ice-sheet dimensions through time. For example, a site that is presently covered by 100 m of ice may have been ice-free during the Holocene, whereas a 400 m thick site farther inland was not; thus, one could better constrain the position of the ice margin during the middle Holocene. Additionally, using the ASIG Drill, there may be enough time in a field season to acquire one or two drill cores from thicker ice sites (e.g., 500–700 m), versus obtaining many drill cores in a single season from ∼ 100 m thick sites using the Winkie Drill. The optimal sampling strategy depends on several factors that relate to a particular scientific objective.
We synthesized the information discussed above to derive a map of candidate areas across the GrIS for drilling (Fig. 3c). While only 3.4 % of the GrIS bed is well suited for subglacial access for the purpose of cosmogenic-nuclide analysis, there are several promising candidate sites: (1) Northwest Greenland, specifically the metamorphic lithologies of the Ellesmere–Inglefield Province in Prudhoe Land–Inglefield Land; (2) two regions in North Greenland – a small area around the head of Victoria Fjord that likely exposes metamorphic lithologies of the Victoria Province and an area adjacent to Mylius-Erichsen Land in eastern North Greenland that contains siliciclastic sedimentary units; (3) Dronning Louise Land in Northeast Greenland, where both crystalline and siliciclastic lithologies are present; and (4) central East Greenland, where the GrIS flows through alpine terrain of mixed lithology en route to the headwaters of the Scoresby Sund, Kong Oscar Fjord and Kejser Franz Joseph Fjord systems. There are additional small areas scattered around the periphery of the GrIS; however, most areas are in alpine-style, ice-field-type settings or lie in small areas between outlet glaciers. In these additional small areas, however, existing uncertainties in available datasets (ice-sheet thickness, basal temperature, etc.) means that drilling there is potentially riskier than in larger patches of the bed that meet drilling requirements.
3.1 Northwest Greenland: Prudhoe Land and Inglefield Land
In Northwest Greenland, the ice sheet in Prudhoe Land and Inglefield Land has broad areas that meet the technical, safety and lithology criteria (Figs. 3c and 5). Here, there are basement rocks consisting of Proterozoic metamorphic lithologies. Proportionally much of the region contains quartz-bearing metamorphic rocks (e.g., paragneiss), albeit with varying quartz content, and in some cases with bands of marble and other potentially non-quartz-bearing units (e.g., syenite, amphibolite; Henriksen et al., 2009). Further, the ice sheet has been surveyed extensively by NASA's Operation IceBridge, and abundant radar data exist. The ice-sheet margin adjacent to Inglefield Land, spanning between the Hiawatha Crater and Prudhoe Dome, is roughly parabolic in profile and rather uniform in velocity, with surface speeds mostly ranging from 3–10 m yr−1. The topography of the ice-sheet bed is low relief, potentially making it difficult to identify small hills and swales where the substrate is less or more likely to host sediment. The landscape fronting the ice is largely bedrock or bedrock overlain by surface blocks either frost-riven or slightly modified by former glaciation. In a few areas, alluvium or glacial deposits exist at the surface. Prudhoe Dome itself (Fig. 5) has a thickness of ∼ 500 m at a summit ridge that rests along a topographic high above a bed elevation of ∼ 800 m a.s.l. The velocities in the summit region of Prudhoe Dome range up to ∼ 20 m yr−1. The Prudhoe Dome summit is a promising place to drill, with a high probability of encountering bedrock at the ice-sheet bed. However, upon deglaciation, the site may maintain local ice isolated from the inland ice, potentially fueled by snowfall due to its proximity to Baffin Bay. Ice-sheet modeling could be used to detect inland (ice sheet) versus peripheral (local) ice survival in locations like this.
3.2 North Greenland: Victoria Fjord
Most of North Greenland is dominated by sedimentary rocks of the lower Paleozoic Franklinian Basin not well suited for providing the hard, quartz-bearing lithologies that work best for in situ cosmogenic-nuclide analysis (Henriksen et al., 2009). At the head of Victoria Fjord (Fig. 6a), however, Henriksen and Jepsen (1985) describe isolated outcrops of crystalline basement in otherwise non-quartz-bearing sedimentary-rock-dominated North Greenland. The crystalline rocks, mostly orthogneiss, comprise several nunataks in Victoria Fjord and additionally crop out in the bottom of two valleys between C. H. Ostenfeld and Ryder glaciers (Fig. 6b). The sedimentary formations consist of Neoproterozoic through Silurian lithologies composed of near-horizontally-bedded shale, siltstone and abundant carbonate units. The outcrop pattern is one of crystalline rocks exposed at lower elevations where the GrIS had eroded away the overlying sub-horizontal sedimentary rocks, or cap rocks. Overall, the outcrop of these quartz-bearing lithologies is promising for their existence at the ice-sheet bed south of the ice-sheet margin. However, because there are topographic highs along the GrIS bed south of the margin, blindly drilling into areas that meet the other technical requirements could lead to encountering cap rocks. For this region, we perform an additional step to estimate where the bed south of the ice margin may be crystalline vs. sedimentary. To project the crystalline/cap rock contact southward under the ice sheet, we use the contact between crystalline rocks and the overlying cap rocks in exposed areas to perform a “three-point problem” – an established method for determining the strike and dip of a plane based on geologic outcrop patterns. As observed by Henriksen and Jepsen (1985), the contact dips gently to the north, and the plane that we calculated in ArcGIS confirms this. Our estimation reveals crystalline rocks outcropping in topographic low areas and cap rocks outcropping in topographic high areas (Fig. 6b). Our estimated contact is simplistic, as there may be folding and faulting that limit the accuracy of this extrapolation. However, this solution provides a straightforward estimate for where crystalline rocks may exist at the ice–bed interface near the head of Victoria Fjord. Using this information, along with Operation IceBridge flight lines and in combination with areas that meet the other technical and safety requirements, indicates promising areas to drill to the southwest of the onset zone of C. H. Ostenfeld Glacier (Fig. 6b).
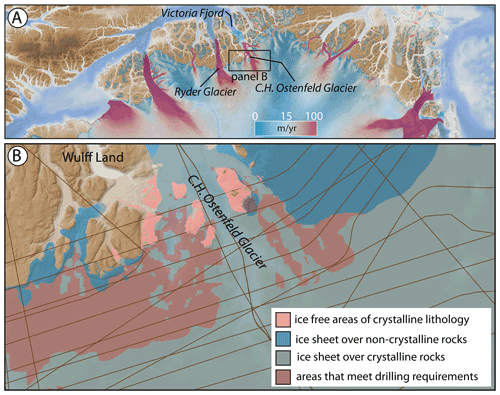
Figure 6(a) North Greenland showing ice-sheet surface velocity; velocity from Greenland Ice Sheet velocity map from Sentinel-1, winter campaign 2019/2020 (version 1.3); QGreenland v2.0. (b) Areas that meet drilling requirements with a focus on bedrock lithology: bright blue are areas under the ice sheet with non-quartz-bearing lithologies, whereas the more muted blue colors depict our estimate of where there are quartz-bearing lithologies at the ice bed. Pink areas are quartz-bearing lithologies beyond the ice margin, and shown in muted red color are the areas that meet the drilling requirements. NASA Operation IceBridge (OIB) flight lines are shown as thin brown lines. Basemap topography and bathymetry from Morlighem et al. (2017).
3.3 North Greenland: Mylius-Erichsen Land
In eastern North Greenland there is an ∼ 100 km stretch of ice margin in Mylius-Erichsen Land (Fig. 7) that lies over quartz-bearing sedimentary lithologies of the Proterozoic Independence Fjord Group (Henriksen et al., 2009). The rocks in this region contain near-horizontally-bedded siltstones, sandstones, and quartzites intruded by Mesoproterozoic dolerite sills, dikes and stocks. Significant portions of the ice sheet in this region meet the technical and safety requirements for drilling, so the suitability for developing GrIS histories rests mostly on the likelihood of encountering preferred lithologies. The pattern on the geologic map and the unit description of the rock formations in the area indicate that the abundance of mafic intrusions means that drilling has a reasonable chance of encountering non-quartz-bearing lithologies. The region has relatively sparse radar lines that cross drilling-suitable areas compared to other parts of Greenland, narrowing the choices of drill sites that have tight constraints on ice thickness and bed shape.
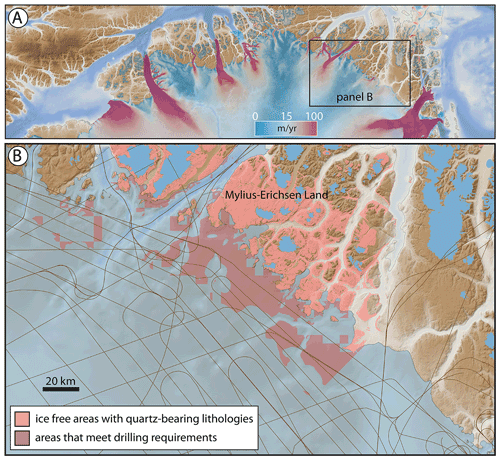
Figure 7(a) North Greenland showing ice-sheet surface velocity; velocity from Greenland Ice Sheet velocity map from Sentinel-1, winter campaign 2019/2020 (version 1.3); QGreenland v2.0. (b) Mylius-Erichsen Land showing areas that meet drilling requirements, with pink areas representing quartz-bearing sedimentary formations (with mafic intrusives) beyond the ice margin. NASA Operation IceBridge (OIB) flight lines are shown as thin brown lines. Basemap topography and bathymetry from Morlighem et al. (2017).
3.4 Northeast Greenland: Dronning Louise Land
The nunatak region of Dronning Louise Land, Northeast Greenland, contains broad areas that meet the technical requirements for subglacial drilling (Fig. 8). The bedrock geology is part of the Caledonian fold belt and contains abundant structures that formed during the Caledonian orogeny (Ordovician–Devonian) leading to the juxtaposition of crystalline and younger sedimentary rock formations in a complicated map pattern (Henriksen et al., 2009; Strachan et al., 1994). The broadest regions that meet the technical criteria and are most favorable for drilling lie on the western (inland) portion of the coastal mountain ranges. Here, nunataks exist 25–30 km west of the coastal mountains and provide information on the bedrock geology most relevant to potential drilling areas. The lithologies are similar to and have been correlated with the Independence Fjord Group found in Mylius-Erichsen Land. Specifically, the local unit that comprises inland nunataks (the Trekant Series) consists largely of quartzitic and feldspathic sandstone and conglomerate with intercalated siltstone and mudstone. Bedrock mapping in the nearby coastal mountains also reveals Mesoproterozoic dolerite intrusions. Sparse radar data limit potential drill sites with close constraints on ice thickness and bed shape. Yet, the region does have potential for tapping into quartz-bearing units, and due to its proximity to the Northeast Greenland Ice Stream, sub-ice cosmogenic-nuclide analyses from the area could yield important constraints on Northeast Greenland Ice Stream history. Finally, the possibility that high-elevation areas remain glaciated by local ice after inland ice recedes should not be ignored. Many of the sub-ice drilling targets are > 1000 m a.s.l., near twentieth-century snowline elevations. To reduce the chances of drilling a site that is occupied by local ice once inland ice recedes, one could assess snowline elevation gradients using the presence/absence of ice caps in peripheral mountains along this coastline. Preliminary analysis shows that snowline elevations increase inland. Extrapolating these gradients to sub-ice areas suitable for drilling could help to guide drill-site selection by identifying sites with elevations lower than projected snowline altitudes. In this way, targeting lower-elevation parts of the sub-ice terrain could be advantageous given the goal of monitoring GrIS history. Additional radar surveys over key areas would be useful for tightening constraints on ice thickness and bed topography over the frozen-bedded patches of Dronning Louise Land.
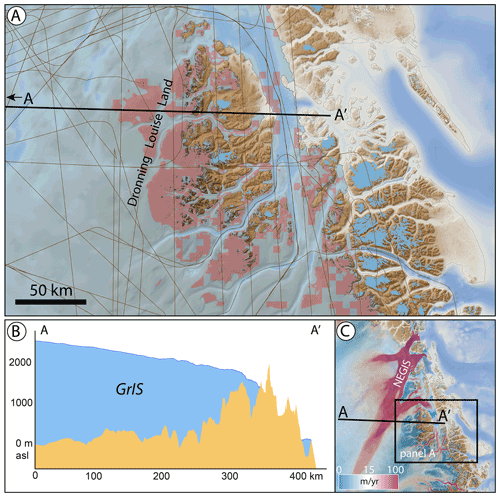
Figure 8(a) Dronning Louise Land showing areas that meet drill requirements; NASA Operation IceBridge (OIB) flight lines are shown as thin brown lines. (b) Topographic profile of bed and GrIS surface from Bed Machine v3 (Morlighem et al., 2017); cross-section line shown in panel (c). (c) NE Greenland with surface velocity showing Northeast Greenland Ice Stream (NEGIS) and location of panel (a); velocity from Greenland Ice Sheet velocity map from Sentinel-1, winter campaign 2019/2020 (version 1.3); QGreenland v2.0. Basemap topography and bathymetry from Morlighem et al. (2017).
3.5 East Greenland
A final place to highlight is central East Greenland, where – similar to Dronning Louise Land – the GrIS abuts and flows through alpine terrain. The western (inland) flank of these mountains has dozens of isolated areas that meet the technical requirements of drilling to the bed (Fig. 9). Like the other areas throughout East and Northeast Greenland, the bedrock geology is highly variable. The headwaters of the Scoresby Sund, Kong Oscar Fjord and Kejser Franz Joseph Fjord systems have a complicated geology relating to the Caledonian orogeny, consisting of Paleoproterozoic crystalline metamorphic and sedimentary formations that are in turn slightly metamorphosed (Henriksson et al., 2009). In terms of finding quartz-bearing rocks most suitable for cosmogenic-nuclide analysis, the region is heterogeneously made up of quartz-bearing (e.g., orthogneiss) and non-quartz-bearing formations (various fine-grained siliciclastic lithologies with occasional mafic intrusions). Inland nunataks provide knowledge of bedrock geology most proximal to potential drill locations and are largely composed of pelitic lithologies (e.g., metamorphosed mudstones). Looking closely at nunatak lithologies reveals some westernmost nunataks of orthogneiss composition, such as inland of J. L. Mowinckel Land (Fig. 9), making the areas in this region that meet the technical requirement promising. A consideration with the potential drilling locations in central East Greenland, again, is the likelihood that they are deglaciated with the recession of inland ice, as opposed to retaining local ice cover. Airborne radar data are also sparse there, so care would be needed to select sites with the best constraints of ice thickness and bed shape.
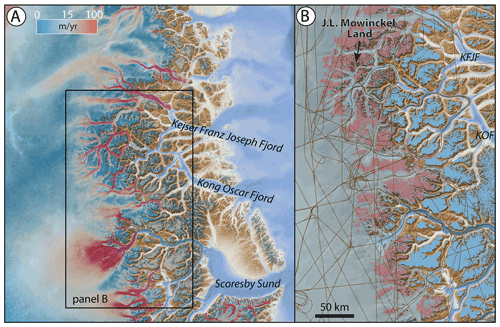
Figure 9(a) East Greenland showing GrIS flowing through alpine terrain; surface velocity highlights major outlets; velocity from Greenland Ice Sheet velocity map from Sentinel-1, winter campaign 2019/2020 (version 1.3); QGreenland v2.0. (b) Portion of East Greenland that includes the most areas that meet the technical requirements of drilling to the bed. Basemap topography and bathymetry from Morlighem et al. (2017).
The purpose of this study is to identify potential targets for subglacial drilling with cosmogenic-nuclide analysis in mind. We find that only 3.4 % of the GrIS is well suited for cosmogenic-nuclide analysis of bed materials using existing drills available from the US Ice Drilling Program and highlight five promising locations in northern and eastern Greenland. Future advances in drill capability, such as the ability to drill through thicker or wet-based ice, would significantly increase the area available for drilling (Fig. 2).
In addition to obtaining drill cores of rock or sediment from the ice-sheet bed, samples of other basal material would also benefit the research community. Basal ice is valuable for (1) measuring trace gases to obtain basal ice age (Bender et al., 2010; Yau et al., 2016b), (2) detrital cosmogenic-nuclide analysis of its mineral component (Bierman et al., 2014), and (3) ancient DNA and biomarkers in organic compounds (Willerslev et al., 2007). Boreholes themselves that are the product of drilling can be instrumented, resulting in direct measurements of basal heat flux values that would provide additional constraints on the basal thermal state of the GrIS (e.g., MacGregor et al., 2022; Colgan et al., 2021) and the history of the Iceland hotspot (e.g., Rogozhina et al., 2016). Finally, precise sampling at the ice–bed interface could lead to the discovery of ancient soils that plausibly exist in areas targeted for drilling that are frozen bedded for long periods. Such samples may be useful for a variety of studies including ancient DNA, macrofossil and biomarker analyses. Additionally, with proper precautions, the uppermost few millimeters of the bed can be preserved in light-free conditions and used to measure for luminescence dating, providing an additional chronometer of past ice-sheet presence/absence (e.g., Christ et al., 2021).
Pairing sub-ice cosmogenic-nuclide analysis with ice-sheet modeling is an important step (Spector et al., 2018). Ice-sheet model simulations have the ability to scale information from single drill sites, or transects of sites, to the entire GrIS. Likewise, results from ice-sheet modeling can help identify which potential drill sites are most sensitive to overall ice-sheet mass balance, thus helping to prioritize sites or to assemble a strategically chosen group of sites. Finally, high-resolution ice-sheet models with fine meshes in areas of peripheral mountainous topography could help with local-ice-survival issues that could complicate cosmogenic-nuclide records from areas where alpine topography is smothered by the GrIS.
In our companion paper (Keisling et al., 2022), we use an ensemble of ice-sheet simulations to illustrate deglaciation styles around the GrIS. The results reveal how much sea level equivalent the GrIS has lost as each perimeter site becomes ice-free, many of which are reachable by the ASIG Drill. The geometry of ice-sheet retreat depends on a number of ice-sheet model parameters, including climate forcing, lapse rate, model initialization, and lithosphere response, among others. We found that some locations become ice-free after a similar amount of ice loss regardless of the uncertainty in these parameters, whereas other locations experience a range of ice-cover histories depending on the model parameters. Our results demonstrate how numerical models can provide another tool to guide site selection by identifying locations where bedrock-derived evidence for ice-free conditions tells us something concrete about ice-sheet size and volume. More observational data of past GrIS change, such as cosmogenic-nuclide analyses, will improve the model-based estimates by identifying the deglaciation styles that are the most realistic, thereby constraining parametric uncertainty. In turn, as models become more competent, they have the ability to scale single drill-site (or transects of sub-ice drill sites) information into a broader picture of regional, or whole, GrIS change. As both of these tools improve, taking an integrated approach offers the greatest potential for leveraging new breakthroughs into societally relevant information about ice-sheet history and stability.
In summary, we consider this study, and ideally drilling efforts taking place in one or more of these candidate sites, as only one of several next steps in the exploration of the GrIS bed and in providing useful data for improving ice-sheet models. We recommend development of drills that can penetrate thicker ice and potentially ice where the bed is thawed. This could be done by modifying existing drill technology (e.g., Timoney et al., 2020; Goodge et al., 2021) or by requiring the development of entirely new drills. Expanding the area of the GrIS available for subglacial drilling would broaden the range of scientific questions that could be addressed regarding GrIS history and the range of possible targets. The application of cosmogenic-nuclide analysis of subglacial materials could then move beyond constraining GrIS history during periods when it is only slightly smaller (∼ 90 %) than its present configuration to constraining times of significant reduction (∼ < 10 %). Additionally, there would be more resolving power for a fuller range of scientific questions, such as what shape the GrIS takes during past interglacials (Plach et al., 2018; Domingo et al., 2020), where ice dynamics may influence large-scale retreat (Aschwanden et al., 2019), or where there are packages of subglacial lake sediments (e.g., Keisling et al., 2020; Paxman et al., 2021) or unique geologic structures (Kjær et al., 2018; MacGregor et al., 2019). Evolving drilling techniques and analyses like this pave the way for targeted exploration of subglacial bed environments, a new frontier in ice-sheet and sea level science.
The geospatial maps illustrated in this paper can be found in the Supplement.
The supplement related to this article is available online at: https://doi.org/10.5194/tc-16-3933-2022-supplement.
JPB led the analysis and writing. CKW led geographic-information-system computations. JPB, JMS, NEY, BAK and SA developed the project design. KP provided strain-rate data. JAM provided basal-thermal-state data. MRA, TK and GB provided information on drills and drilling requirements. All authors contributed to discussions that resulted in the ideas and analysis presented in this paper, and all authors contributed to writing and presentation.
At least one of the (co-)authors is a member of the editorial board of The Cryosphere. The peer-review process was guided by an independent editor, and the authors also have no other competing interests to declare.
Publisher’s note: Copernicus Publications remains neutral with regard to jurisdictional claims in published maps and institutional affiliations.
We thank Greg Balco and an anonymous referee for important suggestions that helped to improve this paper. This work was funded by NSF grants 1933938 (Jason P. Briner) and (Nicolás E. Young, Joerg M. Schaefer, Benjamin A. Keisling).
This research has been supported by the National Science Foundation (grant nos. 1933938 and 1933927).
This paper was edited by Florence Colleoni and reviewed by Greg Balco and one anonymous referee.
Albert, M. R., Slawny, K. R., Boeckmann, G. V., Gibson, C. J., Johnson, J. A., Makinson, K., and Rix, J.: Recent innovations in drilling in ice, in: Advances in Terrestrial and Extraterrestrial Drilling: Ground, Ice, and Underwater, edited by: Bar-Cohen, Y. and Zacny, K., CRC Press, ISBN 9780367674861, 2021.
Aschwanden, A., Fahnestock Mark, A., Truffer, M., Brinkerhoff Douglas, J., Hock, R., Khroulev, C., Mottram, R., and Khan, S. A.: Contribution of the Greenland Ice Sheet to sea level over the next millennium, Science Advances, 5, eaav9396, https://doi.org/10.1126/sciadv.aav9396, 2019.
Balco, G.: Glacier Change and Paleoclimate Applications of Cosmogenic-Nuclide Exposure Dating, Annu. Rev. Earth Pl. Sc., 48, 21–48, https://doi.org/10.1146/annurev-earth-081619-052609, 2020.
Balter-Kennedy, A., Young, N. E., Briner, J. P., Graham, B. L., and Schaefer, J. M.: Centennial- and Orbital-Scale Erosion Beneath the Greenland Ice Sheet Near Jakobshavn Isbræ, J. Geophys. Res.-Earth, 126, e2021JF006429, https://doi.org/10.1029/2021JF006429, 2021.
Bender, M. L., Burgess, E., Alley, R. B., Barnett, B., and Clow, G. D.: On the nature of the dirty ice at the bottom of the GISP2 ice core, Earth Planet. Sc. Lett., 299, 466–473, https://doi.org/10.1016/j.epsl.2010.09.033, 2010.
Bierman, P. R., Corbett, L. B., Graly, J. A., Neumann, T. A., Lini, A., Crosby, B. T., and Rood, D. H.: Preservation of a Preglacial Landscape Under the Center of the Greenland Ice Sheet, Science, 344, 402–405, https://doi.org/10.1126/science.1249047, 2014.
Bierman, P. R., Shakun, J. D., Corbett, L. B., Zimmerman, S. R., and Rood, D. H.: A persistent and dynamic East Greenland Ice Sheet over the past 7.5 million years, Nature, 540, 256–260, https://doi.org/10.1038/nature20147, 2016.
Boeckmann, G. V., Gibson, C. J., Kuhl, T. W., Moravec, E., Johnson, J. A., Meulemans, Z., and Slawny, K.: Adaptation of the Winkie Drill for subglacial bedrock sampling, Ann. Glaciol., 62, 109–117, 2021.
Briner, J. P., Miller, G. H., Davis, P. T., and Finkel, R. C.: Cosmogenic radionuclides from fiord landscapes support differential erosion by overriding ice sheets, GSA Bulletin, 118, 406–420, https://doi.org/10.1130/B25716.1, 2006.
Briner, J. P., Stewart, H. A. M., Young, N. E., Philipps, W., and Losee, S.: Using proglacial-threshold lakes to constrain fluctuations of the Jakobshavn Isbræ ice margin, western Greenland, during the Holocene, Quaternary Sci. Rev., 29, 3861–3874, https://doi.org/10.1016/j.quascirev.2010.09.005, 2010.
Briner, J. P., Lifton, N. A., Miller, G. H., Refsnider, K., Anderson, R., and Finkel, R.: Using in situ cosmogenic 10Be, 14C, and 26Al to decipher the history of polythermal ice sheets on Baffin Island, Arctic Canada, Quat. Geochronol., 19, 4–13, https://doi.org/10.1016/j.quageo.2012.11.005, 2014.
Briner, J. P., Cuzzone, J. K., Badgeley, J. A., Young, N. E., Steig, E. J., Morlighem, M., Schlegel, N.-J., Hakim, G. J., Schaefer, J. M., Johnson, J. V., Lesnek, A. J., Thomas, E. K., Allan, E., Bennike, O., Cluett, A. A., Csatho, B., de Vernal, A., Downs, J., Larour, E., and Nowicki, S.: Rate of mass loss from the Greenland Ice Sheet will exceed Holocene values this century, Nature, 586, 70–74, https://doi.org/10.1038/s41586-020-2742-6, 2020.
Christ, A. J., Bierman, P. R., Schaefer, J. M., Dahl-Jensen, D., Steffensen, J. P., Corbett, L. B., Peteet, D. M., Thomas, E. K., Steig, E. J., Rittenour, T. M. and Tison, J. L.: A multimillion-year-old record of Greenland vegetation and glacial history preserved in sediment beneath 1.4 km of ice at Camp Century, P. Natl. Acad. Sci. USA, 118, e2021442118, https://doi.org/10.1073/pnas.2021442118, 2021.
Cluett, A. A. and Thomas, E. K.: Summer warmth of the past six interglacials on Greenland, P. Natl. Acad. Sci. USA, 118, e2022916118, https://doi.org/10.1073/pnas.2022916118, 2021.
Colgan, W., MacGregor, J. A., Mankoff, K. D., Haagenson, R., Rajaram, H., Martos, Y. M., Morlighem, M., Fahnestock, M. A., and Kjeldsen, K. K.: Topographic Correction of Geothermal Heat Flux in Greenland and Antarctica, J. Geophys. Res.-Earth, 126, e2020JF005598, https://doi.org/10.1029/2020JF005598, 2021.
Dawes, P. R.: The bedrock geology under the Inland Ice: the next major challenge for Greenland mapping, GEUS Bulletin, 17, 57–60, https://doi.org/10.34194/geusb.v17.5014, 2009.
DeConto, R. M., Pollard, D., Alley, R. B., Velicogna, I., Gasson, E., Gomez, N., Sadai, S., Condron, A., Gilford, D. M., Ashe, E. L., Kopp, R. E., Li, D., and Dutton, A.: The Paris Climate Agreement and future sea-level rise from Antarctica, Nature, 593, 83–89, https://doi.org/10.1038/s41586-021-03427-0, 2021.
de Vernal, A. and Hillaire-Marcel, C.: Natural Variability of Greenland Climate, Vegetation, and Ice Volume During the Past Million Years, Science, 320, 1622–1625, https://doi.org/10.1126/science.1153929, 2008.
Domingo, D., Malmierca-Vallet, I., Sime, L., Voss, J., and Capron, E.: Using Ice Cores and Gaussian Process Emulation to Recover Changes in the Greenland Ice Sheet During the Last Interglacial, J. Geophys. Res.-Earth, 125, e2019JF005237, https://doi.org/10.1029/2019JF005237, 2020.
Dyer, B., Austermann, J., D'Andrea William, J., Creel Roger, C., Sandstrom Michael, R., Cashman, M., Rovere, A., and Raymo Maureen, E.: Sea-level trends across The Bahamas constrain peak last interglacial ice melt, P. Natl. Acad. Sci. USA, 118, e2026839118, https://doi.org/10.1073/pnas.2026839118, 2021.
Edwards, T. L., Nowicki, S., Marzeion, B., Hock, R., Goelzer, H., Seroussi, H., Jourdain, N. C., Slater, D. A., Turner, F. E., Smith, C. J., and McKenna, C. M.: Projected land ice contributions to twenty-first-century sea level rise, Nature, 593, 74–82, 2021.
Goelzer, H., Huybrechts, P., Loutre, M.-F., and Fichefet, T.: Last Interglacial climate and sea-level evolution from a coupled ice sheet–climate model, Clim. Past, 12, 2195–2213, https://doi.org/10.5194/cp-12-2195-2016, 2016.
Goelzer, H., Nowicki, S., Payne, A., Larour, E., Seroussi, H., Lipscomb, W. H., Gregory, J., Abe-Ouchi, A., Shepherd, A., Simon, E., Agosta, C., Alexander, P., Aschwanden, A., Barthel, A., Calov, R., Chambers, C., Choi, Y., Cuzzone, J., Dumas, C., Edwards, T., Felikson, D., Fettweis, X., Golledge, N. R., Greve, R., Humbert, A., Huybrechts, P., Le clec'h, S., Lee, V., Leguy, G., Little, C., Lowry, D. P., Morlighem, M., Nias, I., Quiquet, A., Rückamp, M., Schlegel, N.-J., Slater, D. A., Smith, R. S., Straneo, F., Tarasov, L., van de Wal, R., and van den Broeke, M.: The future sea-level contribution of the Greenland ice sheet: a multi-model ensemble study of ISMIP6, The Cryosphere, 14, 3071–3096, https://doi.org/10.5194/tc-14-3071-2020, 2020.
Goodge, J. W. and Severinghaus, J. P.: Rapid Access Ice Drill: a new tool for exploration of the deep Antarctic ice sheets and subglacial geology, J. Glaciol., 62, 1049–1064, https://doi.org/10.1017/jog.2016.97, 2016.
Goodge, J. W., Severinghaus, J. P., Johnson, J., Tosi, D., and Bay, R.: Deep ice drilling, bedrock coring and dust logging with the Rapid Access Ice Drill (RAID) at Minna Bluff, Antarctica, Ann. Glaciol., 62, 324–339, 2021.
Granger, D. E., Lifton, N. A., and Willenbring, J. K.: A cosmic trip: 25 years of cosmogenic nuclides in geology, GSA Bulletin, 125, 1379–1402, https://doi.org/10.1130/B30774.1, 2013.
Hatfield, R. G., Reyes, A. V., Stoner, J. S., Carlson, A. E., Beard, B. L., Winsor, K., and Welke, B.: Interglacial responses of the southern Greenland ice sheet over the last 430 000 years determined using particle-size specific magnetic and isotopic tracers, Earth Planet. Sc. Lett., 454, 225–236, https://doi.org/10.1016/j.epsl.2016.09.014, 2016.
Henriksen, N. and Jepsen, H. F.: Precambrian crystalline basement at the head of Victoria Fjord, North Greenland, Rapport Grønlands Geologiske Undersøgelse, 126, 11–16, https://doi.org/10.34194/rapggu.v126.7907, 1985.
Henriksen, N., Higgins, A. K., Kalsbeek, F., and Pulvertaft, T. C. R.: Greenland from Archaean to Quaternary. Descriptive text to the 1995 Geological map of Greenland, 1 : 2 500 000, 2nd edn., GEUS Bulletin, 18, 1–126, https://doi.org/10.34194/geusb.v18.4993, 2009.
Jordan, T. M., Williams, C. N., Schroeder, D. M., Martos, Y. M., Cooper, M. A., Siegert, M. J., Paden, J. D., Huybrechts, P., and Bamber, J. L.: A constraint upon the basal water distribution and thermal state of the Greenland Ice Sheet from radar bed echoes, The Cryosphere, 12, 2831–2854, https://doi.org/10.5194/tc-12-2831-2018, 2018.
Joughin, I., Das, S. B., Flowers, G. E., Behn, M. D., Alley, R. B., King, M. A., Smith, B. E., Bamber, J. L., van den Broeke, M. R., and van Angelen, J. H.: Influence of ice-sheet geometry and supraglacial lakes on seasonal ice-flow variability, The Cryosphere, 7, 1185–1192, https://doi.org/10.5194/tc-7-1185-2013, 2013.
Keisling, B. A., Nielsen, L. T., Hvidberg, C. S., Nuterman, R., and DeConto, R. M.: Pliocene–Pleistocene megafloods as a mechanism for Greenlandic megacanyon formation, Geology, 48, 737–741, https://doi.org/10.1130/G47253.1, 2020.
Keisling, B. A., Schaefer, J. M., DeConto, R. M., Briner, J. P., Young, N. E., Walcott, C., Winckler, G., Balter-Kennedy, A., and Anandakrishnan, S.: Greenland ice sheet vulnerability under diverse climatic warming scenarios, Earth ArXiv [preprint], https://doi.org/10.31223/X5Q05T, 2022.
Kjær, K. H., Larsen, N. K., Binder, T., Bjørk, A. A., Eisen, O., Fahnestock, M. A., Funder, S., Garde, A. A., Haack, H., Helm, V., and Houmark-Nielsen, M.: A large impact crater beneath Hiawatha Glacier in northwest Greenland, Science Advances, 4, eaar8173, https://doi.org/10.1126/sciadv.aar8173, 2018.
Knutz, P. C., Newton, A. M. W., Hopper, J. R., Huuse, M., Gregersen, U., Sheldon, E., and Dybkjær, K.: Eleven phases of Greenland Ice Sheet shelf-edge advance over the past 2.7 million years, Nat. Geosci., 12, 361–368, https://doi.org/10.1038/s41561-019-0340-8, 2019.
Kuhl, T., Gibson, C., Johnson, J., Boeckmann, G., Moravec, E., and Slawny, K.: Agile Sub-Ice Geological (ASIG) Drill development and Pirrit Hills field project, Ann. Glaciol., 62, 53–66, https://doi.org/10.1017/aog.2020.59, 2021.
Kulessa, B., Hubbard, A. L., Booth, A. D., Bougamont, M., Dow, C. F., Doyle, S. H., Christoffersen, P., Lindbäck, K., Pettersson, R., Fitzpatrick, A. A., and Jones, G. A.: Seismic evidence for complex sedimentary control of Greenland Ice Sheet flow, Science Advances, 3, e1603071, https://doi.org/10.1126/sciadv.1603071, 2017.
Li, J., Paden, J., Leuschen, C., Rodriguez-Morales, F., Hale, R. D., Arnold, E. J., Crowe, R., Gomez-Garcia, D., and Gogineni, P.: High-Altitude Radar Measurements of Ice Thickness Over the Antarctic and Greenland Ice Sheets as a Part of Operation IceBridge, IEEE T. Geosci. Remote, 51, 742–754, https://doi.org/10.1109/TGRS.2012.2203822, 2012.
MacGregor, J. A., Fahnestock, M. A., Catania, G. A., Aschwanden, A., Clow, G. D., Colgan, W. T., Gogineni, S. P., Morlighem, M., Nowicki, S. M., Paden, J. D., and Price, S. F.: A synthesis of the basal thermal state of the Greenland Ice Sheet, J. Geophys. Res.-Earth, 121, 1328–1350, 2016.
MacGregor, J. A., Bottke Jr, W. F., Fahnestock, M. A., Harbeck, J. P., Kjær, K. H., Paden, J. D., Stillman, D. E., and Studinger, M.: A Possible Second Large Subglacial Impact Crater in Northwest Greenland, Geophys. Res. Lett., 46, 1496–1504, https://doi.org/10.1029/2018GL078126, 2019.
MacGregor, J. A., Fahnestock, M. A., Colgan, W. T., Larsen, N. K., Kjeldsen, K. K., and Welker, J. M.: The age of surface-exposed ice along the northern margin of the Greenland Ice Sheet, J. Glaciol., 66, 667–684, https://doi.org/10.1017/jog.2020.62, 2020.
MacGregor, J. A., Chu, W., Colgan, W. T., Fahnestock, M. A., Felikson, D., Karlsson, N. B., Nowicki, S. M. J., and Studinger, M.: GBaTSv2: a revised synthesis of the likely basal thermal state of the Greenland Ice Sheet, The Cryosphere, 16, 3033–3049, https://doi.org/10.5194/tc-16-3033-2022, 2022.
McNabb, R. W., Hock, R., O'Neel, S., Rasmussen, L. A., Ahn, Y., Braun, M., Conway, H., Herreid, S., Joughin, I., Pfeffer, W. T., Smith, B. E., and Truffer, M.: Using surface velocities to calculate ice thickness and bed topography: a case study at Columbia Glacier, Alaska, USA, J. Glaciol., 58, 1151–1164, https://doi.org/10.3189/2012JoG11J249, 2012.
Morlighem, M., Rignot, E., Seroussi, H., Larour, E., Ben Dhia, H., and Aubry, D.: A mass conservation approach for mapping glacier ice thickness, Geophys. Res. Lett., 38, L19503, https://doi.org/10.1029/2011GL048659, 2011.
Morlighem, M., Williams, C. N., Rignot, E., An, L., Arndt, J. E., Bamber, J. L., Catania, G., Chauché, N., Dowdeswell, J. A., Dorschel, B., Fenty, I., Hogan, K., Howat, I., Hubbard, A., Jakobsson, M., Jordan, T. M., Kjeldsen, K. K., Millan, R., Mayer, L., Mouginot, J., Noël, B. P. Y., O'Cofaigh, C., Palmer, S., Rysgaard, S., Seroussi, H., Siegert, M. J., Slabon, P., Straneo, F., van den Broeke, M. R., Weinrebe, W., Wood, M., and Zinglersen, K. B.: BedMachine v3: Complete Bed Topography and Ocean Bathymetry Mapping of Greenland From Multibeam Echo Sounding Combined With Mass Conservation, Geophys. Res. Lett., 44, 11051–11061, https://doi.org/10.1002/2017GL074954, 2017.
Nishiizumi, K., Finkel, R. C., Ponganis, K. V., Graf, T., Kohl, C. P., and Marti, K.: In situ produced cosmogenic nuclides in GISP2 rock core from Greenland summit, in: Fall Meeting 1996, 15–19 December 1996, San Francisco, USA, Eos, Transactions of the American Geophysical Union, 77, F428, abstract OS41B-10, 1996.
Otto-Bliesner, B. L., Marshall, S. J., Overpeck, J. T., Miller, G. H., Hu, A., and CAPE Last Interglacial Project members: Simulating Arctic climate warmth and icefield retreat in the last interglaciation, Science, 311, 1751–1753, 2006.
Paxman, G. J. G., Austermann, J., and Tinto, K. J.: A fault-bounded palaeo-lake basin preserved beneath the Greenland Ice Sheet, Earth Planet. Sc. Lett., 553, 116647, https://doi.org/10.1016/j.epsl.2020.116647, 2021.
Pedersen, M., Weng, W. L., Keulen, N., and Kokfelt, T. F.: A new seamless digital 1 : 500 000 scale geological map of Greenland, GEUS Bulletin, 28, 65–68, https://doi.org/10.34194/geusb.v28.4727, 2013.
Plach, A., Nisancioglu, K. H., Le clec'h, S., Born, A., Langebroek, P. M., Guo, C., Imhof, M., and Stocker, T. F.: Eemian Greenland SMB strongly sensitive to model choice, Clim. Past, 14, 1463–1485, https://doi.org/10.5194/cp-14-1463-2018, 2018.
Poinar, K. and Andrews, L. C.: Challenges in predicting Greenland supraglacial lake drainages at the regional scale, The Cryosphere, 15, 1455–1483, https://doi.org/10.5194/tc-15-1455-2021, 2021.
Reeh, N., Oerter, H., and Thomsen, H. H.: Comparison between Greenland ice-margin and ice-core oxygen-18 records, Ann. Glaciol., 35, 136–144, 2002.
Reyes, A. V., Carlson, A. E., Beard, B. L., Hatfield, R. G., Stoner, J. S., Winsor, K., Welke, B., and Ullman, D. J.: South Greenland ice-sheet collapse during Marine Isotope Stage 11, Nature, 510, 525–528, https://doi.org/10.1038/nature13456, 2014.
Robinson, A., Alvarez-Solas, J., Calov, R., Ganopolski, A., and Montoya, M.: MIS-11 duration key to disappearance of the Greenland ice sheet, Nat. Commun., 8, 16008, https://doi.org/10.1038/ncomms16008, 2017.
Rogozhina, I., Petrunin, A. G., Vaughan, A. P. M., Steinberger, B., Johnson, J. V., Kaban, M. K., Calov, R., Rickers, F., Thomas, M., and Koulakov, I.: Melting at the base of the Greenland ice sheet explained by Iceland hotspot history, Nat. Geosci., 9, 366–369, https://doi.org/10.1038/ngeo2689, 2016.
Scambos, T. A., Bell, R. E., Alley, R. B., Anandakrishnan, S., Bromwich, D. H., Brunt, K., Christianson, K., Creyts, T., Das, S. B., DeConto, R., Dutrieux, P., Fricker, H. A., Holland, D., MacGregor, J., Medley, B., Nicolas, J. P., Pollard, D., Siegfried, M. R., Smith, A. M., Steig, E. J., Trusel, L. D., Vaughan, D. G., and Yager, P. L.: How much, how fast?: A science review and outlook for research on the instability of Antarctica's Thwaites Glacier in the 21st century, Global Planet. Change, 153, 16–34, https://doi.org/10.1016/j.gloplacha.2017.04.008, 2017.
Schaefer, J. M., Finkel, R. C., Balco, G., Alley, R. B., Caffee, M. W., Briner, J. P., Young, N. E., Gow, A. J., and Schwartz, R.: Greenland was nearly ice-free for extended periods during the Pleistocene, Nature, 540, 252–255, https://doi.org/10.1038/nature20146, 2016.
Sommers, A. N., Otto-Bliesner, B. L., Lipscomb, W. H., Lofverstrom, M., Shafer, S. L., Bartlein, P. J., Brady, E. C., Kluzek, E., Leguy, G., Thayer-Calder, K., and Tomas, R. A.: Retreat and Regrowth of the Greenland Ice Sheet During the Last Interglacial as Simulated by the CESM2-CISM2 Coupled Climate–Ice Sheet Model, Paleoceanography and Paleoclimatology, 36, e2021PA004272, https://doi.org/10.1029/2021PA004272, 2021.
Spector, P., Stone, J., Cowdery, S. G., Hall, B., Conway, H., and Bromley, G.: Rapid early-Holocene deglaciation in the Ross Sea, Antarctica, Geophys. Res. Lett., 44, 7817–7825, https://doi.org/10.1002/2017GL074216, 2017.
Spector, P., Stone, J., Pollard, D., Hillebrand, T., Lewis, C., and Gombiner, J.: West Antarctic sites for subglacial drilling to test for past ice-sheet collapse, The Cryosphere, 12, 2741–2757, https://doi.org/10.5194/tc-12-2741-2018, 2018.
Strachan, R. A., Friderichsen, J. D., Holdsworth, R. E., and Jepsen, H. F.: Regional geology and Caledonian structure, Dronning Louise Land, North-East Greenland, Rapport Grønlands Geologiske Undersøgelse, 162, 71–76, 1994.
Sugden, D. E.: Glacial Erosion by the Laurentide Ice Sheet, J. Glaciol., 20, 367–391, https://doi.org/10.3189/S0022143000013915, 1978.
The IMBIE team: Mass balance of the Antarctic Ice Sheet from 1992 to 2017, Nature, 558, 219–222, https://doi.org/10.1038/s41586-018-0179-y, 2018.
The IMBIE team: Mass balance of the Greenland Ice Sheet from 1992 to 2018, Nature, 579, 233–239, https://doi.org/10.1038/s41586-019-1855-2, 2020.
Timoney, R., Worrall, K., Firstbrook, D., Harkness, P., Rix, J., Ashurst, D., Mulvaney, R., and Bentley, M. J.: A low resource subglacial bedrock sampler: The percussive rapid access isotope drill (P-RAID), Cold Reg. Sci. Tech., 177, 103113, https://doi.org/10.1016/j.coldregions.2020.103113, 2020.
Willerslev, E., Cappellini, E., Boomsma, W., Nielsen, R., Hebsgaard, M. B., Brand, T. B., Hofreiter, M., Bunce, M., Poinar, H. N., Dahl-Jensen, D., and Johnsen, S.: Ancient Biomolecules from Deep Ice Cores Reveal a Forested Southern Greenland, Science, 317, 111–114, https://doi.org/10.1126/science.1141758, 2007.
Yau, A. M., Bender, M. L., Blunier, T., and Jouzel, J.: Setting a chronology for the basal ice at Dye-3 and GRIP: Implications for the long-term stability of the Greenland Ice Sheet, Earth Planet. Sc. Lett., 451, 1–9, https://doi.org/10.1016/j.epsl.2016.06.053, 2016a.
Yau, A. M., Bender, M. L., Robinson, A., and Brook, E. J.: Reconstructing the last interglacial at Summit, Greenland: Insights from GISP2, P. Natl. Acad. Sci. USA, 113, 9710–9715, https://doi.org/10.1073/pnas.1524766113, 2016b.
Young, N. E., Lesnek, A. J., Cuzzone, J. K., Briner, J. P., Badgeley, J. A., Balter-Kennedy, A., Graham, B. L., Cluett, A., Lamp, J. L., Schwartz, R., Tuna, T., Bard, E., Caffee, M. W., Zimmerman, S. R. H., and Schaefer, J. M.: In situ cosmogenic 10Be–14C–26Al measurements from recently deglaciated bedrock as a new tool to decipher changes in Greenland Ice Sheet size, Clim. Past, 17, 419–450, https://doi.org/10.5194/cp-17-419-2021, 2021.