the Creative Commons Attribution 4.0 License.
the Creative Commons Attribution 4.0 License.
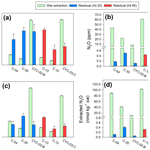
Brief Communication: The reliability of gas extraction techniques for analysing CH4 and N2O compositions in gas trapped in permafrost ice wedges
Ji-Woong Yang
Go Iwahana
Sangyoung Han
Kyungmin Kim
Alexander Fedorov
Methane (CH4) and nitrous oxide (N2O) compositions in ground ice may provide information on their production mechanisms in permafrost. However, existing gas extraction methods have not been well tested. We tested conventional wet and dry gas extraction methods using ice wedges from Alaska and Siberia, finding that both methods can extract gas from the easily extractable parts of the ice (e.g. gas bubbles) and yield similar results for CH4 and N2O mixing ratios. We also found insignificant effects of microbial activity during wet extraction. However, both techniques were unable to fully extract gas from the ground ice, presumably because gas molecules adsorbed onto or enclosed in soil aggregates are not easily extractable. Estimation of gas production in a subfreezing environment of permafrost should consider such incomplete gas extraction.
- Article
(1194 KB) - Full-text XML
-
Supplement
(1244 KB) - BibTeX
- EndNote
Permafrost preserves large amounts of soil carbon (C) and nitrogen (N) in a frozen state (e.g. Hugelius et al., 2014; Salmon et al., 2018), temporarily removing this frozen C and N from active global cycles. Therefore, future projections of permafrost stability are of great interest, particularly because thawing permafrost may lead to decomposition and/or remineralisation of the buried soil C and N and their abrupt emission into the atmosphere in the form of greenhouse gases (GHGs) such as carbon dioxide (CO2), methane (CH4), and nitrous oxide (N2O), which in turn can trigger positive feedbacks (e.g. Salmon et al., 2018). In addition, projected polar amplification (e.g. Masson-Delmotte et al., 2013) may strengthen these positive feedbacks. However, the processes responsible for in situ C and N remineralisation and GHG production in ground ice are poorly understood, despite the fact that ground ice accounts for a substantial portion (up to ∼40–90 % by volume) of Pleistocene ice-rich permafrost, or Yedoma (e.g. Kanevskiy et al., 2013; Jorgenson et al., 2015).
The gases trapped in ground ice allow unique insights into the origin of ground ice and evidence for in situ microbial aerobic and anaerobic respiration (Boereboom et al., 2013; Kim et al., 2019; Lacelle et al., 2011). Among others, the GHGs in ground ice may provide detailed information on in situ biogeochemical processes responsible for GHG production (i.e. methanogenesis, nitrification, and denitrification) (e.g. Boereboom et al., 2013; Kim et al., 2019). However, the relevant analytical methods remain poorly scrutinised. Boereboom et al. (2013) utilised the conventional melting–refreezing method (wet extraction) used in polar ice core analyses, in which the ice samples were melted under a vacuum to liberate the enclosed gases and then refrozen to expel the dissolved gases present in the meltwater. Other studies conducted by Russian scientists used an on-site melting method, in which a large (1–3 kg) block of ground ice sample was melted in a saturated sodium chloride (NaCl) solution in order to minimise gas dissolution (Arkhangelov and Novgorodova, 1991). A recent study instead used a dry extraction technique to prevent microbial activity during wet extraction (Kim et al., 2019), which employed a needle crusher in a vacuum to crush approximately 10 g of ice sample without melting (Shin, 2014).
In this study, for the first time, we tested the reliability of both wet and dry extraction methods for CH4 and N2O mixing ratios and contents (volume or moles of gas in a unit mass at standard temperature and pressure (STP) conditions) using permafrost ground ice samples. Ice-wedge samples from Alaskan and Siberian permafrost were used because ice wedges are one of the most abundant morphological features of massive ground ice, consisting of approximately 5–50 % by volume of the upper permafrost (Kanevskiy et al., 2013; Jorgenson et al., 2015). More specifically, we aimed to address the following scientific questions: (1) do wet and dry extraction methods yield different results? (2) Are the melting–refreezing results affected by microbial activity during gas extraction? (3) How effectively does the wet/dry extraction extract gases from ice wedges? To address the first question, we compared CH4 and N2O results from dry and wet extractions. For the second question, we applied the wet extraction method to both biocide-treated and control samples. Finally, for the third question we carried out tests with and without extended needle system hits in a crushing chamber, as well as additional dry extraction from ice samples that had been degassed by our wet extraction method.
2.1 Ice samples and sample preparation
The ice-wedge samples used in this study were collected from Churapcha, Cyuie (central Yakutia), and Zyryanka (northeastern Yakutia) in Siberia, as well as from northern Alaska (Fig. S1 in the Supplement). The Churapcha site (61.97∘ N, 132.61∘ E) was located approximately 180 km east of Yakutsk, while the Cyuie site (61.73∘ N, 130.42∘ E) was located approximately 30 km southeast of Yakutsk. The Cyuie samples were collected from two outcrops (CYB and CYC) (Kim et al., 2019). At each site, 30 cm long ice-wedge cores were drilled perpendicular to the outcrop surface (Figs. S2 and S3).
Zyryanka is located in the southern boreal region of the Kolyma River, at the junction of the Chersky and Yukaghir ranges, in a region affected by thermokarst development (Fedorov et al., 1991). Site A (Zy-A) was located on a tributary of the Kolyma River, ∼22 km north of Zyryanka. Site B (Zy-B) was ∼14 km west of the start of the Kolyma tributary, which begins ∼11 km north of Zyryanka. Site F (Zy-F) was located ∼4 km west of the tributary that leads to site B. The ground ice samples were collected from riverbank walls exposed by lateral erosion using a chainsaw (Fig. S4). Most of the outcrops that were sampled for ground ice were on the first (lowest) terrace of the river.
For the Alaskan sampling locations, Bluff03 (69.40∘ N, 150.95∘ W) and Bluff06 (69.14∘ N, 150.61∘ W) were located in the Alaska North Slope region, ∼120 and ∼150 km from the Arctic Ocean, or ∼100 and ∼70 km northwest of the Toolik Field Station (68.63∘ N, 149.59∘ W), respectively. Samples from Bluff03 were collected by chainsaw from bluff walls that had developed by gully formation on a gentle slope of the Yedoma. Samples from Bluff06 were collected from outcrops within eroded frozen peatland in a thaw lake basin (Fig. S5). All ice-wedge samples used in this study were stored in a chest freezer at ∘C before analysis.
Ice-wedge ice is different from polar ice cores in that its gas mixing ratios are not homogeneous (e.g. Kim et al., 2019), which may hinder exact comparison with results from adjacent ice samples. We therefore randomly mixed subsamples to reduce the effect of the heterogeneous gas composition distribution (the “random cube” method, hereafter). Approximately 100–200 g of an ice-wedge sample was cut into 25–50 cubes of 3–4 g each; for each experiment, ∼10–12 cubes were randomly chosen so that the total weight of the subsample was ∼40 g.
2.2 Gas extraction procedures
2.2.1 Dry extraction (needle crusher)
For dry extraction, we used a needle-crusher system at Seoul National University (SNU, Seoul, South Korea) (Shin, 2014). In brief, 8–13 g of ice sample was crushed in a cold vacuum chamber (extraction chamber). The ice samples were usually hit five times by the needle set. The temperature within the extraction chamber was maintained at −37 ∘C using a cold ethanol-circulating chiller. The extracted gas was dried by passing it through a water vapour trap at −85 ∘C and cryogenically trapping it in a stainless-steel tube (sample tube) at approximately −257 ∘C using a helium closed-cycle refrigerator (He-CCR). Since the extraction chamber cannot accommodate ∼40 g of ice at once, the ∼40 g of random cube subsamples were extracted using three sequential extractions and the gas liberated from each extraction was trapped in a sample tube.
Following extraction, the sample tubes were detached from the He-CCR, warmed to room temperature (∼20 ∘C), and attached to a gas chromatograph (GC) equipped with an electron capture detector (ECD) and a flame ionisation detector (FID) to determine the mixing ratios of CH4 and N2O. Details of the GC system are given in Ryu et al. (2018). The daily calibration curves were established using working standards of 15.6±0.2 ppm CH4, 10 000±30 ppm CH4, 2960±89 ppb N2O, 29 600±888 ppb N2O, and a modern air sample from a surface firn at Styx Glacier, Antarctica (obtained in November 2016), which was calibrated as 1758.6±0.6 ppb CH4 and 324.7±0.3 ppb N2O by the National Oceanic and Atmospheric Administration (NOAA).
2.2.2 Wet extraction (melt–refreeze)
For the control and HgCl2-treated wet extraction experiments, a melting–refreezing wet extraction system at SNU was employed (Yang et al., 2017; Ryu et al., 2018). The gas extraction procedure was identical to the procedure described in Yang et al. (2017) and Ryu et al. (2018), except for the sample gas trapping procedure (see below). Ice-wedge subsamples of ∼40 g (composed of 10–12 ice cubes for each) were placed in a glass container welded to a stainless-steel flange (sample flask), and the laboratory air inside the sample flasks was evacuated for 40 min. The sample flasks were then submerged in a warm (∼50 ∘C) tap water bath to melt the ice samples. After melting was complete, the meltwater was refrozen by chilling the sample flasks with cold ethanol (below −70 ∘C). The sample gas in the headspace of each sample flask was then expanded to the volume-calibrated vacuum line to estimate the volume of extracted gas and trapped in a stainless-steel sample tube by the He-CCR device. We attached the He-CCR device to our wet extraction line, and the gas samples in the flasks were cryogenically trapped. There were two reasons for using He-CCR instead of direct expansion to a GC: (1) to better compare the dry and wet extraction methods by applying the same trapping procedure, and (2) to maximise the amount of sample gas for GC analysis, because gas expansion from a large flask allows only a small fraction of gas to be measured by the GC.
For biocide-treated tests, 1.84 mmol of mercuric chloride (HgCl2) was applied per unit kilogram of soil, following established procedures for soil sterilisation (Fletcher and Kaufman, 1980). We obtained the average dry soil mass (0.33 g) from the leftover meltwater samples of the previous wet extractions, which were carried out for comparison between dry and wet extractions. Taking this into account, we added 24 µL of saturated HgCl2 solution (at 20 ∘C) to the sample flasks. The flasks with HgCl2 solution were then frozen in a deep freezer at ∘C to prevent the dissolution of ambient air into the solution during ice sample loading. After the wet extraction procedure was complete, the extracted gas was trapped in a sample tube and the CH4 and N2O mixing ratios were determined using the same GC–ECD–FID system as the dry-extracted gas. The resulting CH4 and N2O mixing ratios were not corrected for partial dissolution in ice melt in the flasks, because CH4 and N2O trapped in refrozen ice were negligible compared to the ranges of the systematic blanks (see Appendix).
2.3 Gas content
The analytical methods described previously were used to determine the mixing ratios of CH4 and N2O in the extracted gas. Converting these mixing ratios into moles of CH4 and N2O per unit mass of ice-wedge sample (CH4 and N2O content, respectively, hereafter) required data on the amount of gas extracted. As the gas content is a measure of gas volume enclosed in a unit mass of ice sample at STP (in ), the CH4 and N2O contents can be calculated using the gas content, the total mass of the random cube ice, and the gas mixing ratio. The gas content in the control and HgCl2-treated wet extraction experiments was calculated from the temperature and pressure of the extracted gas and the internal volume of the vacuum line. The details of the extraction system and correction methods used for estimating gas content are described in Yang (2019). Similarly, the gas content of the dry extraction samples was also inferred from the volume and pressure of gas inside the vacuum line once the sample tube was attached to the line for GC analysis. The uncertainties of the calculated CH4 and N2O contents were calculated by using the error propagation of the blanks and gas content uncertainties (see Appendix for uncertainty estimation of the blank corrections and gas contents).
2.4 Dry soil content
Dry soil content was measured using the leftover meltwater from the control wet extraction tests. After these were complete, the sample flasks were shaken thoroughly and the meltwater samples were each poured into a 50 mL conical tube. The meltwater and soils were separated by a centrifugal separator at 3000 rpm for 10 min. The separated wet soils were wind-dried in evaporating dishes at ∼100 ∘C for 24 h. The weight of each individual evaporating dish was pre-measured before use. The dry soil content was calculated by subtracting the weight of the evaporating dish from the total weight of the dried soil sample plus the evaporating dish.
3.1 Comparison between wet and dry extraction methods
The results from the wet and dry extractions were compared using 23 CH4 and 21 N2O ice-wedge samples from Alaska and Siberia. In both the CH4 and N2O mixing ratio analyses, we found that the wet and dry extraction results did not differ significantly (p>0.1), regardless of sampling site or soil content (Fig. 1a–d). We note that the heterogeneous distribution of gas mixing ratios at the centimetre scale (Kim et al., 2019) may not have been completely smoothed out by our subsample selection, although we randomly chose 8–12 ice cubes for each measurement. Some previous studies have avoided using the wet extraction method because of potential reactivation of microbial CH4 and/or N2O production in ice melt (e.g. Cherbunina et al., 2018; Kim et al., 2019). Assuming that activation of microbial metabolism is unlikely during dry extraction at a temperature of −37 ∘C in the extraction chamber for <1 h, our findings may imply that wet extraction does not stimulate microbial reactivation to a measurable extent.
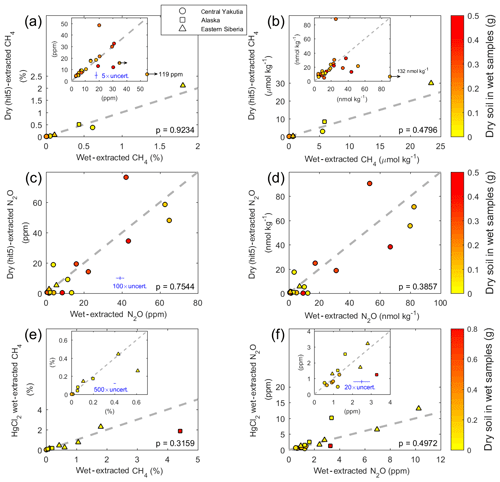
Figure 1Comparison of CH4 and N2O mixing ratios and contents obtained by different extraction methods. Shown are scatter plots between wet and dry (hit5) extraction results of CH4 (a and b) and N2O (c and d), and between control and biocide-treated wet extraction results for CH4 (e) and N2O (f). The “hit5” denotes the dry extraction with five hits (see Sect. 3.3). Left panels (a, c, and e) and panel (f) present the mixing ratios of gas in bubbles, while panels (b) and (d) in the right column present moles of gas in a unit mass of ice (gas content). The sampling locations are indicated by different symbols. The colour of each data point indicates the dry soil weight in the subsamples used in control wet extraction. The 1σ uncertainties of the mixing ratios (a, c, e, and f) are magnified by 5×, 20×, 100×, and 500× as denoted as blue error bars (see Appendix). The error bars are not visible where the error bars are smaller than markers. The grey dashed lines are 1:1 reference lines. Note that the units of the axes of the insets in (e) and (f) are identical to the original plots. The p value of the two-sided Students t test of each comparison is denoted at the bottom right corner of each plot.
3.2 Testing microbial alteration during wet extraction
To test the microbial production of CH4 and N2O during wet extraction more accurately, we conducted wet extraction experiments on samples treated with HgCl2, a commonly used effective biocide (e.g. Torres et al., 2005), and compared the results with those of untreated (control) wet extractions. We prepared 12 additional ice-wedge samples using the random cube method for these tests (see Sect. 2). We found no significant differences between the control and HgCl2-treated wet extraction results for both CH4 and N2O mixing ratios (Fig. 1e and f), indicating that the bias due to microbial activity during ∼1 h of the melting–refreezing procedure was not significant. This was further supported by tests on an additional 12 ice-wedge samples (using the random cube protocol) treated with 2-bromoethanesulfonate (BES), a specific methanogenesis inhibitor (e.g. Nollet et al., 1997) (Fig. A3). Similar to the HgCl2-treated experiments, 25 µL of a saturated BES solution was added to each sample flask. These additional tests were carried out only for CH4. The two-sided t test for the CH4 data indicated an insignificant difference between the two results (p>0.9). Data from individual sampling sites also did not show significant differences (p>0.9 for the Alaskan samples and p>0.5 for the central Yakutian samples).
According to microbial sequencing studies that have shown the presence of viable microbes in permafrost and ground ice (e.g. Katayama et al., 2007), it is likely that culturable microbes exist in the ice-wedge samples used in this study. However, considering that at least 14 d and up to 3 months of culturing was required to identify microbe colonies extracted from ground ice (Katayama et al., 2007; Lacelle et al., 2011), our melt–refreeze time of an hour was insufficient for microbial activity to resume production of CH4 and N2O.
3.3 Dry extraction efficiency and gas mixing ratios
One limitation of our needle crushing dry extraction technique was the inability to completely extract gas from ice samples, because small ice particles and/or flakes placed in the space between the needles were not fully crushed. The gas extraction efficiency of the SNU needle-crusher system has been reported as ∼80–90 % for polar ice core samples (Shin, 2014). However, the gas extraction efficiency has not been tested for ice-wedge samples. Depending on the extraction efficiency, the needle crushing method could underestimate the gas contents if the gas is not completely extracted. Another possible bias in the gas mixing ratios arises if the CH4 and N2O compositions are different between the crushed and uncrushed portions of the ice-wedge samples.
To estimate the biases arising from incomplete gas extraction, we designed a series of tests to identify the differences of the CH4 and N2O mixing ratios and contents between the crushed and uncrushed sample portions. Each randomly collected ice-wedge sample was first crushed by the regular dry extraction procedure (by hitting it five times with the needle system, “hit5”), and the gas liberated from the sample was trapped in a sample tube. We then performed an additional 100 hits on the leftover ice (“hit100”), monitored the amount of additional gas liberated, and trapped the additional gas in a separate sample tube. Comparisons between the hit5 and hit100 results are summarised in Fig. 2 and Table A1.
We regarded the ratio of gas content of hit100 to that of hit5 (hit100 / hit5 ratio, hereafter) as a measure of the gas extraction efficiency of the needle-crusher system. The results demonstrate an average hit100 / hit5 ratio of gas content of 0.40±0.07 for the Zyryanka samples, 0.24±0.07 for the Bluff samples, and 0.14±0.11 for the Cyuie samples (Table A1). Despite the fact that the number of samples was limited, the ice-wedge samples from the different sites showed distinct hit100 / hit5 ratios of the amount of extracted gas. However, we observed that the leftover ice from the Bluff and Zyryanka samples was not well crushed, even after 100 hits with the needle crusher. This was especially true if the ice subsamples contained soil aggregates: in these cases the frozen soil aggregates were barely crushed. In contrast, the Cyuie samples were relatively well crushed, and the leftover samples were apparently finer-sized ice flakes. We also observed that the hit100 / hit5 ratios of gas content were highly variable within samples from a particular site, implying that the extraction efficiency of the needle crusher depended not only on-site characteristics but also on the individual ice sample hardness. When compared with the dry soil content measured from the subsamples used for wet extraction, no relationship was observed between the dry soil content and the extraction efficiency (Figs. 1 and A3). In addition, in the case of samples uncrushed by the hit100 test, it was difficult to estimate the extraction efficiency using the hit100 / hit5 ratio of gas content, as the hit100 tests liberated only a marginal portion of gas from these samples. This was because the large-sized uncrushed soil aggregates or particles may have prohibited the needle crusher from crushing the small-sized ice flakes or grains. The needles move up and down together as they are fixed to a pneumatic linear motion feedthrough device, so if there is a sizeable soil clod that cannot be crushed, it blocks the needle crusher from moving further down. Therefore, we do not recommend using a needle-crusher system to measure gas contents in ice-wedge samples.
The hardness of the ice samples may also affect the gas mixing ratio analysis in the hit5 and hit100 procedures. The hit100 / hit5 ratios of the CH4 mixing ratio of the Bluff and Zyryanka samples were less than one in four out of six samples, yielding an average of 0.9±0.5. However, all five samples from the Cyuie ice wedges had ratios greater than 1, with an average of 4.7±2.6 (Table A1). The higher hit100 / hit5 ratio of CH4 mixing ratios of Cyuie samples indicated that the gases extracted via the hit100 procedure had higher CH4 mixing ratios than the gases extracted via the hit5 procedure. Considering these results with those discussed previously, we speculate that there are three ways gas can be trapped in ice-wedge ice: enclosed in bubbles, adsorbed on soil particles, and entrapped in soil aggregates. The better-crushed leftover ice flakes in the Cyuie samples may have allowed most of the gas in bubbles and part of the CH4 molecules adsorbed on soil particles and/or trapped in microsites within soil aggregates to be liberated. Thus, the hit5 CH4 mixing ratios of the Cyuie samples may have better reflected the gas mixing ratios in bubbles, while the hit100 results reflected more of the contribution from gas adsorbed on soil and trapped within soil aggregates because the ice sample containing larger-sized aggregates had greater hardness than those with smaller aggregates or fine particles. If this was the case for the Cyuie samples, we can infer that CH4 is more concentrated in soil particles and in microsites within soil aggregates than in bubbles in the ice. This is partly supported by evidence that ice-wedge layers exhibit relatively trace amounts of CH4 compared to the surrounding permafrost soil layers (Rivkina et al., 2007); however, this needs to be further evaluated by detailed microbial and chemical analyses. Meanwhile, in the Bluff and Zyryanka samples, the hit5 results reflected the mixing ratios of the gases from the crushed portions, regardless of their origin: bubbles, particle adsorption, or microsites in aggregates (Fig. 2 and Table A1). Given that some of the Bluff and Zyryanka ice-wedge samples were not fully crushed by the hit100 tests, additional hits or another extraction technique may have been required. Unlike CH4, the N2O mixing ratios from the hit100 extractions were higher than the hit5 in 10 of 11 samples, regardless of the sampling site. The hit100 / hit5 ratios of N2O mixing ratios of the Bluff and Zyryanka samples (1.9±0.8 on average) were not significantly different (p=0.32) from those of the Cyuie samples (2.9±1.8 on average). This can probably be explained by the fact that the N2O mixing ratio is not necessarily higher in soil-rich ice because N2O is an intermediate product of denitrification, while CH4 is produced as the final product of methanogenesis.
Although a different crushing technique might be more suitable for ice-wedge samples, none of the existing dry extraction techniques – centrifugal ice microtome (Bereiter et al., 2013), mechanical grater (Etheridge et al., 1988), or ball-mill crusher (Schaefer et al., 2011) – is more advantageous for ice-wedge analysis than the needle-crusher system used in this study. The hard portion of ice wedges (e.g. frozen soil aggregates and large soil particles) could easily damage the metal blades of the centrifugal ice microtome and mechanical grater devices, or block the space within the ball-mill chamber, limiting the movement of the milling balls.
It is worth noting that friction between stainless-steel surfaces could produce CH4 with carbon from the damaged surface and hydrogen gas (Higaki et al., 2006). If needle crushing causes contamination in this way, the dry extraction results should be affected by the number of hits. To check the impact of the needle crushing procedure on ice-wedge CH4 and N2O measurements, we carried out blank tests by changing the number of hits from 5 to 100. The results of these tests showed no systematic offset among the experiments with different numbers of hits (Fig. A2), implying that the crushing procedure did not affect the dry extraction results for CH4 and N2O. Even though minor contamination did occur, its effects had already been subtracted via blank correction and taken into account in the overall error estimation (see Appendix). Therefore, we concluded that our findings were not artefacts of metal friction during crushing.
To summarise, from the hit5 and hit100 comparison tests, we found that (1) the needle-crusher method was not able to fully crush the ice-wedge ice samples and thus was unsuitable for measuring gas content in a unit mass of ice, and (2) weak crushing (e.g. a small number of hits by the needle-crusher system) may better reflect gas mixing ratios in the soft parts of the samples (such as air bubbles) than strong crushing (e.g. a greater number of hits).
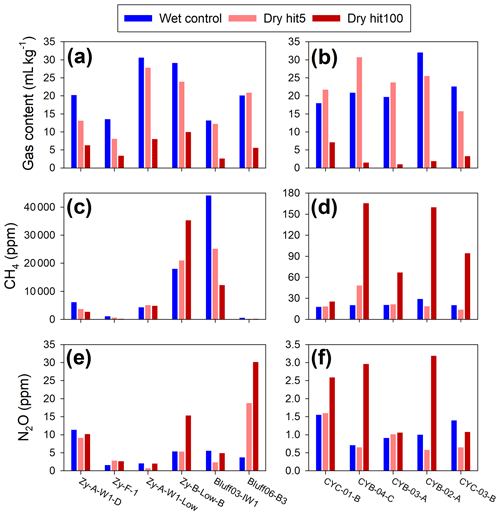
Figure 2Results of dry extraction tests with 5 and an additional 100 hits to ice-wedge samples, denoted as “hit5” and “hit100”, respectively (c–f). Also plotted are gas content results from both experiments, where the hit100 values are given in units of millilitre per kilogram (mL kg−1) at STP conditions (a and b). It should be noted that the hit100 gas content results indicate the additional amount of gas extracted after hit5 crushing and evacuation.
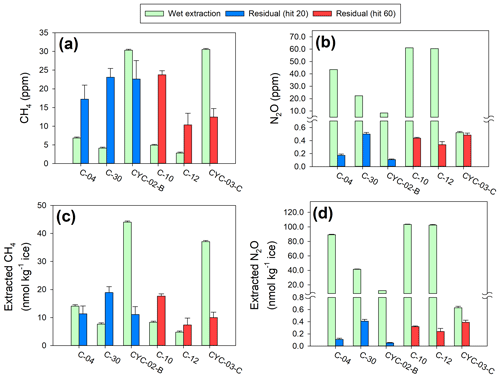
Figure 3Comparison of wet-extracted gas and residual gas for CH4 and N2O mixing ratios (a and b) and contents (c and d). The residual gas was extracted from the dry extraction method using the wet-degassed ice samples. The light green bars show the results of initial wet extraction, and the blue and red bars indicate the dry extraction of wet-degassed ice with 20 and 60 hits, respectively. The Cyuie samples are denoted as “CYC”, while “C” indicates the Churapcha samples.
3.4 Residual gas mixing ratios and content after wet extraction
To examine how well the gas was extracted by wet extraction, we applied the dry extraction method to refrozen ice-wedge samples after wet extraction. We first prepared degassed ice-wedge samples that had undergone repetitive wet extractions (wet-degassed ice, hereafter). Once the wet extraction experiments were completed, we repeated two cycles of melting–refreezing and evacuation procedures to degas the ice melt. After degassing by a total of three cycles of wet extraction and evacuation, the outermost surfaces (∼2 mm) of the wet-degassed ice were trimmed away in the walk-in freezer at SNU on the morning of experiments. The wet-degassed ice was then inserted into the needle crusher, the crusher chamber was evacuated, a specific amount of standard air was injected, and the wet-degassed ice samples were hit 20 or 60 times by the needle crusher. The amount of gas and the gas mixing ratio of the additionally extracted gas from the wet-degassed ice are given in Fig. 3 and Table A2.
These tests using the wet-degassed ice showed an additional gas extraction of ∼12–20 , which was ∼43–88 % of the amount of gas extracted during the initial wet extraction. The additionally extracted gas from the dry extraction is referred to as residual gas hereafter. This was in remarkable contrast to the <1 % residual fraction of the SNU wet extraction system for ice from polar ice sheets. If such a considerable amount of gas was left intact by repeated wet extractions, the composition of the additional gas must be important for understanding the extent of the bias for conventional wet extraction results.
Figure 3 and Table A2 show the mixing ratios and contents of CH4 and N2O in the residual gas. The mixing ratios of the residual gas were estimated using mass balance calculations with observed mixing ratios and the amounts of the injected standard and extracted residual gas. The CH4 mixing ratios of the residual gas ranged from 10.37 to 23.78 ppm, similar to the range of the wet-extracted gas, indicating that CH4 in ice wedges cannot be fully extracted by a melting–refreezing procedure. We suspect two possible reasons for this: (1) during wet extraction, the ice-wedge samples melted and the soil particles settled at the bottom of the sample flask without any physical impact to the soil particles, causing the adsorbed CH4 molecules on the soil particles to remain adsorbed, and (2) during refreezing the soils accumulated at the bottom of the flasks crumpled around the centre of the refrozen ice because the sample flasks were chilled from outside, which facilitated gas entrapment within the frozen soil aggregate. In contrast, the N2O mixing ratios of the residual gas exhibited very low values compared to those from the initial wet extraction (Fig. 3 and Table A2). These results implied that most of the N2O in ice wedges was extracted by three melting–refreezing cycles, such that only a small amount of N2O was left adsorbed or entrapped in ice-wedge soils. We therefore suggest that this might be attributed to the high solubility of N2O to water compared to CH4 (Fogg and Sangster, 2003). However, further investigation is needed to better understand this.
In summary, we found that a certain amount of gas remained in ice wedges, even after three cycles of wet extraction, and that it was extractable instead by needle crushing. This implies that, unlike polar ice cores, wet extraction of ice wedges does not guarantee near-complete gas extraction, and therefore precise measurements of the gas content of ice wedges are difficult to obtain. This difficulty in measuring gas content imposes a large uncertainty when estimating CH4 and N2O contents. Furthermore, we found that the residual gas had a similar-order CH4 mixing ratio to the gas extracted by initial melting–refreezing, indicating that a comparable amount of CH4 still remained unextracted in ice wedges. Hence, a novel extraction method is required to produce reliable gas content and gas mixing ratios in ice wedges. In contrast, our results show that the N2O content of the residual gas was at trace levels, suggesting that most of the N2O in ice wedges is extractable during initial melting–refreezing. Therefore, wet extraction could be applicable for estimating the N2O content of ice wedges. However, given that the above evidence resulted from three consecutive cycles of melting–refreezing and evacuation, it is unclear how many melting–refreezing cycles are required to extract most N2O from ice wedges. It should be noted that combination of repetitive wet extractions with dry extraction does not guarantee reliable estimation of the N2O mixing ratio, because extraction efficiency of the other gas components may be different from that of N2O. Our findings imply that previous estimates of CH4 budgets in ground ice based on the wet extraction principle (e.g. Boereboom et al., 2013; Cherbunina et al., 2018) might have been underestimated, and that CH4 production within subfreezing permafrost environments could be larger than previously estimated. Future study should be devoted to a novel extraction method able to easily and effectively extract gas molecules from ice.
In this study we carried out comparisons between (1) wet and dry extraction, (2) untreated and biocide-treated wet extraction, and (3) gas extraction from the easy-to-extract and difficult-to-extract parts of ice-wedge ice in order to better understand the characteristics of each extraction method and adequately analyse CH4 and N2O mixing ratios and gas contents from permafrost ice wedges. Based on these comparisons, our major findings can be summarised as follows:
-
Existing wet and dry extraction methods allow gas extraction from the soft parts of ice (e.g. ice bubbles) and show insignificant differences in CH4 and N2O mixing ratios.
-
Wet extraction results are unlikely to be affected by microbial production of CH4 and N2O during the melting–refreeze procedure.
-
Both dry and wet extraction methods are not able to fully extract gas from ice-wedge samples, presumably due to gas adsorbed on soil particles or enclosed within soil aggregates, which may have different gas mixing ratios compared to the gas in bubbles. Further research is required to develop a proper method to quantify and extract adsorbed and enclosed gases. In the meantime, we propose that both existing techniques may be suitable for gas mixing ratio measurements for bubbles in relatively soft ice wedges (i.e. easily crushed ice wedges by hit5 extraction, e.g. Cyuie ice wedges in this study). Although the N2O content in ice wedges may be measured by using repeated wet extractions, this is not the case for determining the N2O mixing ratio.
-
Previous estimates of ground ice CH4 and N2O budget may be underestimated, implying that the greenhouse gas production in subfreezing permafrost environments is larger than the current understanding.
-
Saturated NaCl solution is unnecessary for preventing microbial activity during melting, as employed by, for example, Cherbunina et al. (2018). However, it remains an open question how effectively the adsorbed gas molecules can be extracted by this method.
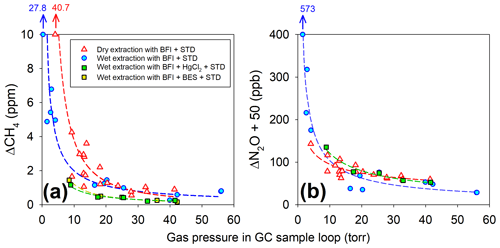
Figure A1Systematic blank of the needle crushing (dry extraction) and melting–refreezing (wet extraction) methods for (a) CH4 and (b) N2O measurements in control and biocide (HgCl2)-treated experiments. Also plotted are the CH4 blanks of BES-treated wet extractions. The dashed lines represent exponential regression curve fittings. Note that all data are plotted against the amount of gas trapped in the sample tube, presented here as the pressure in the GC sample loop when the sample gas is expanded. The grey shaded areas indicate the range of ice-wedge samples used in this study (see main text). The capital-delta (Δ) notation on the y axes indicates the offset from the values of the standard used.
Since the SNU dry extraction systems, including the sample tubes, were originally designed for CO2 measurements from polar ice cores, these systems have not been tested for CH4 and N2O analysis. We therefore carried out a series of tests to estimate the systematic blank, which is defined here as blanks.
The systematic blanks were tested with bubble-free ice (BFI) and standard air in a cylinder calibrated by NOAA. The BFI was prepared as described in Yang et al. (2017), other than cutting the BFI block into small pieces of 3–4 g to mimic the random cube sampling protocol (see Sect. 2). The systematic blanks for the dry extraction method were tested as follows. A total of ∼45 g of BFI cubes were placed into the crushing chamber, which was sealed with a copper gasket and evacuated until the interior gas pressure dropped lower than ∼60 mTorr because of the vapour pressure formed by sublimation of the BFI. After evacuation was completed, standard gas was injected into the crushing chamber. The amount of standard injected was controlled by a volume-calibrated vacuum line in the dry extraction system. Then the BFI samples were hit with the needle system 5–100 times and the gases in the chamber were passed through a water trap and cryogenically pumped into the sample tubes, using the He-CCR. The number of hits did not significantly affect the systematic blank (Fig. A2), and the regression curve for blank correction was fitted to the entire set of data points (red dashed curve in Fig. A1).
For the wet extraction, a total of ∼45 g of BFI cubes were placed into each sample flask. The flasks were connected to the wet extraction line, sealed with a copper gasket, and then evacuated. Once a vacuum was established, a known amount of standard gas was injected into each flask and the flasks were submerged into a warm water bath for ∼40 min to melt completely. The flasks were then submerged into a cold ethanol bath (chilled to −80 ∘C) to refreeze. For the HgCl2- and sodium-2-BES-treated experiments, we first prepared saturated solutions of HgCl2 and BES at room temperature (20 ∘C) and added 24 µL of HgCl2 or 20 µL of BES solution into the empty flasks in a fume hood. Then we placed the flasks in a deep freezer (at −45 ∘C for 20 min) to freeze the solutions before the BFI pieces were placed.
The results of the blank experiments are shown in Fig. A1. The systematic blanks appeared to be inversely correlated with the gas pressure in the sample tube. The systematic blank test results were fitted using exponential regression curves (dashed lines in Fig. A1), and these regression curves were then used for systematic blank correction in our ice-wedge sample analyses.
To calculate uncertainties of the blank corrections, the blank test data were fitted with exponential regression curves (Fig. A1). The root-mean-square deviations (RMSDs) of the data from the regression curves were taken as the uncertainties of blank corrections (Fig. 1). Since the ice-wedge data used in this study showed pressure in the GC sample loop of about 8–50 Torr, the RMSDs were estimated from the blank test data within this pressure range. The uncertainty of the gas content measurement was calculated by error propagation from those of pressure, line volume, and mass of ice samples.
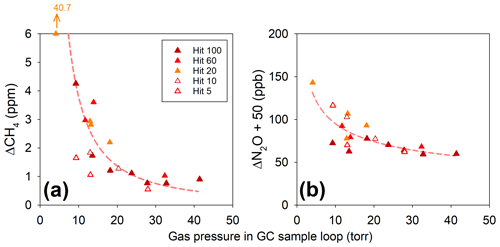
Figure A2Influence of different amounts of hitting on the systematic blank of the needle crushing (dry extraction) system for (a) CH4 and (b) N2O measurements. Note that all data are plotted against the amount of gas trapped in the sample tube, presented here as the pressure in the GC sample loop when the sample gas is expanded (see main text). The capital-delta (Δ) notation on the y axes indicates the offset from the values of the standard used.
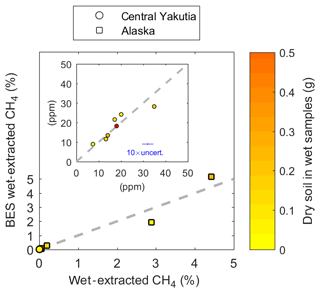
Figure A3Comparison between control and BES-treated wet extraction results for CH4. The sampling area is indicated by different symbols. The colour of each data point indicates the dry soil weight in the subsamples used in control wet extraction. The grey dashed lines are 1:1 reference lines. The blue error bar indicates the 1σ uncertainty of mixing ratios magnified by 10×.
Table A1Results of dry extraction tests with 5 and an additional 100 hits to the ice-wedge samples, denoted as “hit5” and “hit100”, respectively. “hit100∕hit5” is the ratio in extracted gas content or gas mixing ratio of the hit100 to hit5 cases. Also shown are gas content results from both experiments, where the hit100 values are given in units of millilitre per kilogram (mL kg−1) at STP conditions. It should be noted that the hit100 gas content results indicate the additional amount of gas extracted after hit5 crushing and evacuation.
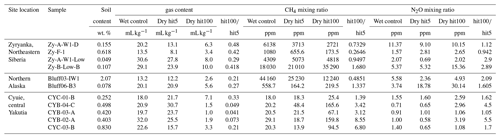
All data used in this study are available at the Zenodo repository: https://doi.org/10.5281/zenodo.3701243 (Yang et al., 2020).
The supplement related to this article is available online at: https://doi.org/10.5194/tc-14-1311-2020-supplement.
JWY and JA conceived the research and designed the experiments. GI, JA, KK, and AF drilled the ice-wedge ice samples from Alaska and Siberia. JWY, JA, SH, and KK conducted the laboratory experiments. JWY and JA led the manuscript preparation with inputs from all other co-authors.
The authors declare that they have no conflict of interest.
The authors greatly acknowledge those who contributed to collect ice-wedge ice samples. We thank Gwangjin Lim and Jaeyoung Park for their help in sample preparations and gas extraction experiments, and Min Sub Sim for kind advice on inhibition experiments for methanogen.
This project was supported by the Basic Science Research Program through the National Research Foundation of Korea (NRF) (grant nos. NRF-2018R1A2B3003256 and NRF-2018R1A5A1024958) and the NASA ABoVE (Arctic Boreal and Vulnerability Experiment (grant no. NNX17AC57A)).
This paper was edited by Ylva Sjöberg and reviewed by two anonymous referees.
Arkhangelov, A. A. and Novgorodova, E. V.: Genesis of massive ice at “Ice Mountains”, Yenesei River, Western Siberia, according to results of gas analyses, Permafrost Periglac. Proc., 2, 167–170, https://doi.org/10.1002/ppp.3430020210, 1991.
Bereiter, B., Stocker, T. F., and Fischer, H.: A centrifugal ice microtome for measurements of atmospheric CO2 on air trapped in polar ice cores, Atmos. Meas. Tech., 6, 251–262, https://doi.org/10.5194/amt-6-251-2013, 2013.
Boereboom, T., Samyn, D., Meyer, H., and Tison, J.-L.: Stable isotope and gas properties of two climatically contrasting (Pleistocene and Holocene) ice wedges from Cape Mamontov Klyk, Laptev Sea, northern Siberia, The Cryosphere, 7, 31–46, https://doi.org/10.5194/tc-7-31-2013, 2013.
Cherbunina, M. Y., Shmelev, D. G., Brouchkov, A. V., Kazancev, V. S., and Argunov, R. N.: Patterns of spatial methane distribution in the upper layers of the permafrost in central Yakutia, Mosc. Univ. Geol. Bull., 73, 100–108, 2018.
Etheridge, D. M., Pearman, G. I., and de Silva, F.: Atmospheric trace-gas variations as revealed by air trapped in an ice core from Law Dome, Antarctica, Ann. Glaciol., 10, 28–33, https://doi.org/10.3189/S0260305500004110, 1988.
Fedorov, A. N., Botulu, T. A., Vasiliev, I. S., Varlamov, S. P., Gribanova, S. P., and Dorofeev, I. V.: Permafrost-landscape map of the Yakut ASSR, Gosgeodezia, Moscow, Russia, Map, 1991 [in Russian].
Fletcher, C. L. and Kaufman, D. D.: Effect of sterilization methods on 3-chloroaniline behavior in soil, J. Agric. Food Chem., 28, 667–671, https://doi.org/10.1021/jf60229a016, 1980.
Fogg, P. G. T., and Sangster, J.: Chemicals in the Atmosphere: Solubility, Sources and Reactivity, John Wiley and Sons, Inc., Chichester, 2003.
Higaki, S., Oya, Y., and Makide, Y.: Emission of methane from stainless steel surface investigated by using tritium as a radioactive tracer, Chem. Lett., 35, 292–293, https://doi.org/10.1246/cl.2006.292, 2006.
Hugelius, G., Strauss, J., Zubrzycki, S., Harden, J. W., Schuur, E. A. G., Ping, C.-L., Schirrmeister, L., Grosse, G., Michaelson, G. J., Koven, C. D., O'Donnell, J. A., Elberling, B., Mishra, U., Camill, P., Yu, Z., Palmtag, J., and Kuhry, P.: Estimated stocks of circumpolar permafrost carbon with quantified uncertainty ranges and identified data gaps, Biogeosciences, 11, 6573–6593, https://doi.org/10.5194/bg-11-6573-2014, 2014.
Jorgenson, M. T., Kanevskiy, M., Shur, Y., Moskalenko, N., Brown, D. R. N., Wickland, K., Striegl, R., and Koch, J.: Role of ground ice dynamics and ecological feedbacks in recent ice wedge degradation and stabilization, J. Geophys. Res., 120, 2280–2297, https://doi.org/10.1002/2015JF003602, 2015.
Kanevskiy, M., Shur, Y., Jorgenson, M. T., Ping, C. -L., Michaelson, G. J., Fortier, D., Stephani, E., Dillon, M., and Tumskoy, V.: Ground ice in the upper permafrost of the Beaufort Sea coast of Alaska, Cold Reg. Sci. Technol., 85, 56–70, https://doi.org/10.1016/j.coldregions.2012.08.002, 2013.
Katayama, T., Tanaka, M., Moriizumi, J., Nakamura, T., Brouchkov, A., Douglas, T. A., Fukuda, M., Tomita, F., and Asano, K.: Phylogenetic analysis of bacteria preserved in a permafrost ice wedge for 25 000 years, Appl. Env. Microbiol., 73, 2360–2363, https://doi.org/10.1128/AEM.01715-06, 2007.
Kim, K., Yang, J. -W., Yoon, H., Byun, E., Fedorov, A., Ryu, Y., and Ahn, J.: Greenhouse gas formation in ice wedges at Cyuie, central Yakutia, Permafrost Periglac. Process., 30, 48–57, https://doi.org/10.1002/ppp.1994, 2019.
Lacelle, D., Radtke, K., Clark, I. D., Fisher, D., Lauriol, B., Utting, N., and Whyte, L. G.: Geomicrobiology and occluded O2-CO2-Ar gas analyses provide evidence of microbial respiration in ancient terrestrial ground ice, Earth Planet. Sci. Lett., 306, 46–54, https://doi.org/10.1016/j.epsl.2011.03.023, 2011.
Masson-Delmotte, V., Schulz, M., Abe-Ouchi, A., Beer, J., Ganopolski, A., Rouco, J. F. G., Jansen, E., Lambeck, K., Luterbacher, J., Naish, T., Osborn, T., Otto-Bliesner, B., Quinn, T., Ramesh, R., Rojas, M., Shao, X., and Timmerman, A.: Information from paleoclimatic archives, Climate change 2013: The Physical science basis, Contribution of working group I to the fifth assessment report of the Intergovernmental Panel on Climate Change, Cambridge: Cambridge University Press, 383–464, https://doi.org/10.1017/CBO9781107415324.013, 2013.
Nollet, L., Demeyer, D., and Verstraete, W.: Effect of 2-bromoethanesulfonic acid and Peptostreptococcus products ATCC 35244 addition on stimulation of reductive acetogenesis in the ruminal ecosystem by selective inhibition of methanogenesis, Appl. Environ. Microbiol., 63, 194–200, 1997.
Rivkina, E., Shcherbakova, V., Laurinavichius, K., Petrovskaya, L., Krivushin, K., Kraev, G., Pecheritsina, S., and Gilichinsky, D.: Biogeochemistry of methane and methanogenic archaea in permafrost, FEMS Microbiol. Ecol., 61, 1–15, https://doi.org/10.1111/j.1574-6941.2007.00315.x, 2007.
Ryu, Y., Ahn, J., and Yang, J. -W.: High-precision measurement of N2O concentration in ice cores, Environ. Sci. Technol., 52, 731–738, https://doi.org/10.1021/acs.est.7b05250, 2018.
Salmon, V. G., Schadel, C., Bracho, R., Pegoraro, E., Celis, G., Mauritz, M., Mack, M. C., and Schuur, E. A. G.: Adding depth to our understanding of nitrogen dynamics in permafrost soils, J. Geophys. Res., 123, 2497–2512, https://doi.org/10.1029/2018JG004518, 2018.
Schaefer, H., Lourantou, A., Chappellaz, J., Luthi, D., Bereiter, B., and Barnola, J.-M.: On the stability of partially clathrated ice for analysis of concentration and δ13C of palaeo-atmospheric CO2, Earth Planet. Sci. Lett., 307, 334–340, https://doi.org/10.1016/j.epsl.2011.05.007, 2011.
Shin, J.: Atmospheric CO2 variations on millennial time scales during the early Holocene, Master thesis, School of Earth and Environmental Sciences, Seoul National University, South Korea, 58 pp., 2014.
Torres, M. E., Mix, A. C., and Rugh, W. D.: Precise δ13C analysis of dissolved inorganic carbon in natural waters using automated headspace sampling and continuous-flow mass spectrometry, Limnol. Oceanogr.-Methods, 3, 349–360, 2005.
Yang, J.-W.: Paleoclimate reconstructions from greenhouse gas and borehole temperature of polar ice cores, and study on the origin of greenhouse gas in permafrost ice wedges, Ph.D. thesis, School of Earth and Environmental Sciences, Seoul National University, Seoul, 188 pp., 2019.
Yang, J.-W., Ahn, J., Brook, E. J., and Ryu, Y.: Atmospheric methane control mechanisms during the early Holocene, Clim. Past, 13, 1227–1242, https://doi.org/10.5194/cp-13-1227-2017, 2017.
Yang, J. -W., Ahn, J., Iwahana, G., Han, S., Kim, K., and Fedorov, A.: Comparison of different gas extraction techniques to analyze CH4 and N2O compositions in gas trapped in permafrost ice wedges, Zenodo, https://doi.org/10.5281/zenodo.3701243, 2020.