the Creative Commons Attribution 4.0 License.
the Creative Commons Attribution 4.0 License.
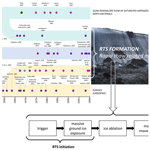
Review article: Retrogressive thaw slump characteristics and terminology
Marina Leibman
Alexander Kizyakov
Hugues Lantuit
Ilya Tarasevich
Ingmar Nitze
Alexandra Veremeeva
Guido Grosse
Retrogressive thaw slumps (RTSs) are spectacular landforms that occur due to the thawing of ice-rich permafrost or melting of massive ground ice, often in hillslope terrain. RTSs occur in the Arctic, the subarctic, and high mountain (Qinghai–Tibet Plateau) permafrost regions and are observed to expand in size and number due to climate warming. As the observation of RTSs is receiving more and more attention due to their important role in permafrost thaw; impacts on topography; mobilization of sediment, carbon, nutrients, and contaminants; and their effects on downstream hydrology and water quality, the thematic breadth of studies increases and scientists from different scientific backgrounds and perspectives contribute to new RTS research. At this point, a wide range of terminologies originating from different scientific schools is used, and we identified the need to provide an overview of variable characteristics of RTSs to clarify terminologies and ease the understanding of the literature related to RTS processes, dynamics, and feedbacks. We review the theoretical geomorphological background of RTS formation and landform characteristics to provide an up-to-date understanding of the current views on terminology and underlying processes. The presented overview can be used not only by the international permafrost community but also by scientists working on ecological, hydrological, and biogeochemical consequences of RTS occurrence and by remote-sensing specialists developing automated methods for mapping RTS dynamics. The review will foster a better understanding of the nature and diversity of RTS phenomena and provide a useful base for experts in the field but also ease the introduction to the topic of RTSs for scientists who are new to it.
- Article
(8801 KB) - Full-text XML
- BibTeX
- EndNote
Permafrost in the Arctic is impacted by thawing in step with ongoing pronounced Arctic warming due to climate change (Biskaborn et al., 2019; Smith et al., 2022). Thaw of ice-rich permafrost results in the formation of characteristic landforms due to sometimes rapid terrain subsidence and erosion. One typical and regionally widespread landform formed by the thaw of ice-rich permafrost or melting of massive ground ice is a slope failure termed retrogressive thaw slump (RTS) (Mackay, 1966). These spectacular landforms in the Northern Hemisphere occur throughout the Arctic, the subarctic, and high mountain regions (Qinghai–Tibet Plateau) with ice-rich permafrost and have a significant environmental impact (Kokelj and Lewkowicz, 1999). Figure 1 shows examples of different RTSs photographed across the Northern Hemisphere. RTSs exhibit regional variations in their appearance and characteristics.
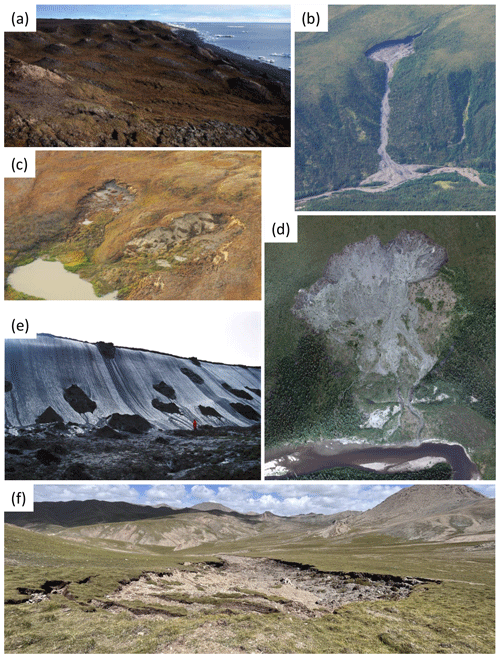
Figure 1Various RTSs across the Northern Hemisphere. (a) Stabilized yedoma RTS on Belkovsky Island, NE Siberia, Russia, September 2002. Photo: Guido Grosse. (b) RTS on the Peel Plateau, NW Canada, July 2023. Photo from airplane: Guido Grosse. (c) Two RTSs on the central Gydan Peninsula, West Siberia, Russia, September 2020. Photo from helicopter: Elena Babkina. (d) RTS in Selawik, Alaska, USA, July 2021. Aerial camera image: AWI. (e) Yedoma RTS in Oyagos Yar, NE Siberia, Russia, September 2002. Photo: Guido Grosse. (f) RTS on the Qinghai–Tibet Plateau, China, August 2023. Photo: Zhuoxuan Xia.
RTS initiation not only alters the topography, hydrology, and vegetation cover but also contributes to substantial sediment, carbon, and nutrient fluxes to downstream environments with impacts on water quality and aquatic ecosystems (Kokelj et al., 2005; Mesquita et al., 2010).
Historically, RTS research started with the first mention of exposed ice in a retrogressive thaw slump probably dating back to 1881 by Dall in his publication on observations in Alaska (Dall, 1881) The first intensive studies on RTSs were conducted much later in the latter half of the 20th century in Canada (Lamothe and St-Onge, 1961; Mackay, 1966; Kerfoot, 1969) and Siberia (Popov et al., 1966; Czudek and Demek, 1970). These studies on RTSs were field-based and focused on ground ice, morphometry, and dynamics. The publications were written either in English or Russian, with different terms applied to these landforms depending on scientific approaches. Unfortunately, the level of knowledge exchange and reciprocal citation among RTS researchers from Canada and the former USSR was relatively low, leading to the establishment of disparate views and terminology for RTS used in the literature.
The strong rise in scientific exchange and international collaborations at the end of the 20th century, including joint expeditions within the permafrost community in general and within the topic of RTS in particular (i.e., Vaikmäe et al., 1993; Ingólfsson, and Lokrantz, 2003; Are et al., 2005), as well as the emergence of remote-sensing methods, substantially broadened the scope of RTS research (Romanenko, 1998; Lantuit and Pollard, 2005; Lantz and Kokelj, 2008; Leibman et al., 2021). Today, a large body of the recent literature predominantly focuses on monitoring RTS activity by measuring retreat rates (Kizyakov et al., 2006; Wang et al., 2009; Laccelle et al., 2010) and volume changes (Kizyakov et al., 2006; Clark et al., 2021; Jiao et al., 2022; Bernhard et al., 2022), identifying driving factors (Harris and Lewkowicz, 2000; Lacelle et al., 2010) or, more generally, the mapping of RTSs (Pollard, 2000; Lipovsky and Huscroft, 2006; Khomutov and Leibman, 2008; Swanson and Hill, 2010; Segal et al., 2016). Recent publications on RTS mapping notably shifted away from a focus on geological and geomorphological aspects to developing advanced methodologies of RTS detection and classification using spatially and/or temporally high-resolution remote-sensing data and digital elevation data, frequently employing artificial intelligence methods (Huang et al., 2020; Nitze et al., 2021; Yang et al., 2023).
However, despite the increasing number of studies and strongly rising interest in RTS among the permafrost and remote-sensing research communities, there is still no commonly agreed terminology on the RTS phenomenon. Various authors apply different terminology to describe the same morphology and processes or use the same terms for different processes. This leads to several difficulties in communication about RTS within and across research communities. First of all, since the terminology is not always clearly defined or translated in the literature, it can lead to potential misunderstandings about what exact features or processes have been investigated in a particular study. The confusion about the object of the study may cause incomparability of the datasets from different RTS studies. Furthermore, different labeling of the same features may result in a completely different image of the phenomena. For example, Nitze et al. (2024) conducted an experiment where 12 domain experts from different countries manually mapped RTSs in Canada and Russia. The results demonstrated a large mismatch of the RTS labeling in Yakutia, Russia, which can partially be explained by different terminology used in the publications describing this region. The confusion in the terminology and labeling of RTSs can also affect the related studies on how RTSs impact hydrology, geochemistry, and ecology or their physical modeling, based on the established terms and concepts in the literature. Moreover, various terms used in the keywords lead to new publications and new data being missed and not included in further reviews.
This work aims to clarify the existing terminology of RTS phenomena and ease the understanding of published studies. The paper presents commonly observed RTS characteristics and a neutral review of existing RTS terminology in the literature. Our review considers a broad variety of RTSs in the Northern Hemisphere.
2.1 Morphometry and dynamics
RTSs can be of various sizes starting from less than 0.1 ha in area and reaching up to ∼ 80 ha, as in the case of the Batagay slump in Yakutia, Russia (Kizyakov et al., 2023). Some authors describe RTSs larger than 5 ha (Kokelj et al., 2015) or larger than 20 ha (Lacelle et al., 2015) as megaslumps. Known RTS headwall (in the meaning of the entire steep wall) heights range from a few meters up to 55 m in the Batagay slump (Kizyakov et al., 2023). RTS headwall length can exceed 1 km, as reported for Yakutia, Russia (Costard et al., 2021).
Reported length-to-width ratios range from below 1 (Lantuit and Pollard, 2008; Ardelean et al., 2020) up to 3 (Niu et al., 2016) and even 5 (Lantuit and Pollard, 2008). Some field studies in Canada suggest that this ratio increases with time due to the headwall retreating faster than the sidewalls, leading to a landform lengthening (Lewkowicz, 1987b). Other studies in Siberia report the widening of RTSs with time due to their merging with neighboring RTSs (Runge et al., 2022; Leibman et al., 2023a).
RTS dynamics can be estimated by measuring headwall retreat rates. Reported RTS headwall retreat rates vary from several centimeters per year on the Qinghai–Tibet Plateau, China (Sun et al., 2017), to up to ∼ 66 m yr−1 estimated for the Yugorsky Peninsula, Russia (Leibman et al., 2021). Similar extreme headwall retreat rates of ∼ 27 m yr−1 were reported for some RTSs in Canada (Lacelle et al., 2015; Jones et al., 2019).
2.2 Position and topography
RTSs form inland or on the coast. Inland RTSs can be found on lake shores, riverbanks, slopes of temporary streams, or valley slopes. As an RTS develops, the thawed material is transferred downstream to valley bottoms or to the nearest water body.
The extent of RTSs appearing at a particular position varies and is strongly controlled by the topographical and geological characteristics of the area. For example, RTSs in mountain regions mostly occur on slopes unrelated to the waterbodies, as on the Qinghai–Tibet Plateau, China (Niu et al., 2012; Huang et al., 2018; Hu et al., 2019), or in the Yana Highlands, East Siberia (Kizyakov et al. 2023), while RTSs in the flat terrain of the Yamal and Gydan peninsulas, West Siberia, are generally found next to lake shores (Nesterova et al., 2021). A first analysis across the Arctic has not revealed any correlations between the influence of RTS position in the terrain and its size or activity so far (Bernhard et al., 2022).
RTSs were found across a wide range of slopes, including on gentle terrain slopes of < 5° (De Krom, 1990; Leibman et al., 2023a), medium slopes of 5 to 10° (Niu et al., 2016), and steep slopes of >10° (Czudek and Demek, 1970; Barry, 1992; Robinson, 2000). Some studies found that RTSs on steeper slopes tend to have higher headwall retreat rates (see Sect. 3.1.1) than those that occur on less steep slopes (Robinson, 2000).
RTSs occur on a great variety of slope aspects. While some studies investigating different regions across the Arctic reported that their observed RTSs tended to have different prevailing slope orientations (Kokelj et al., 2009; Lacelle et al., 2015; Jones et al., 2019; Nesterova et al., 2021; Bernhard et al., 2022), several other studies found that higher RTS ablation rates and headwall retreat (see Sect. 3.1.1) are related to southern aspects (Lewkowicz, 1987a; Grom and Pollard, 2008; Lacelle et al., 2015). However, several other studies did not find any link between the slope aspect and RTS activity (Wang et al., 2009; Nesterova et al., 2021; Bernhard et al., 2022). Bernhard et al. (2022) suggested that differences in the RTS aspect may be explained by regional geological history that defines ice content and ice distribution, which are the main factors of RTS occurrence (Mackay, 1966; Kerfoot, 1969).
2.3 Ground ice
A high excess ground ice content is a prerequisite for RTS occurrence. The shallower the ground ice table, the higher the likelihood that seasonal thawing will reach and start melting the ice, potentially triggering the initiation of the RTS. Regions with abundant ground ice presence in Canada feature widespread and ubiquitous slumps (Lamothe and St-Onge, 1961; Mackay, 1966; Kokelj et al., 2017). Similar observations were reported for central Yamal, Russia (Babkina et al., 2019). RTSs in areas with a thinner ground-ice-rich layer tend to stabilize faster due to the rapid ice exhaustion (Kizyakov, 2005). The type of ground ice and its local distribution can define some morphologic characteristics of RTSs (see Sect. 3.1) and affect retreat rates. For example, RTSs forming in syngenetic-ice-rich Yedoma deposits with polygonal ice wedges are usually accompanied by the presence of baydzherakhs (conical remnant mounds; for details, see Sect. 3.1.6) on the slump floors. De Krom and Pollard (1989) found that, on Herschel Island, Canada, large ice wedges melted more slowly than the enclosing massive ground ice body. While abundant ground ice is necessary for RTS formation it is not the only control for RTS occurrence.
2.4 Triggers
An RTS forms once very ice-rich permafrost or massive ground ice becomes exposed for any reason and starts melting. Triggers of this exposure can be any anthropogenic or natural permafrost disturbances.
Anthropogenic triggers can be any disturbance of the thermal balance of the permafrost due to direct human actions. For example, mining was reported to trigger ice exposure and further formation of RTSs in Canada (Fraser and Burn, 1997) and on the Qinghai–Tibet Plateau, China (Wei et al., 2006; Niu et al., 2012).
Natural triggers can be separated into climatic, geomorphological, and wildfire. Wildfire removes vegetation and possibly the upper protective organic soil layer, leading to a deeper thaw than normal (Harry and MacInnes 1988; Jorgenson and Osterkamp, 2005; Lacelle et al., 2010). Climatic triggers are generally associated with a deepening of the active layer and the subsequent thawing of ice-rich deposits or massive ground ice. It can be caused by
-
unusually long warm-weather periods (Lacelle et al., 2010; Balser et al., 2014; Swanson and Nolan, 2018; Lewkowicz and Way, 2019; Jones et al., 2019) or
-
heavy precipitation and snowmelt events (Leibman et al., 2003; Lamoureux and Lafrenière, 2009; Balser et al., 2014).
Geomorphological triggers slightly differ inland and on the coast. Coastal triggers reported in the literature include
-
thermal erosion (or thermo-erosion) (Burn and Lewkowicz, 1990; Lantuit et al., 2012; Kokelj and Jorgenson, 2013) and
-
coastal erosion (Burn and Lewkowicz, 1990; Burn, 2000; Dallimore et al., 1996; Lantuit et al., 2012; Kokelj and Jorgenson, 2013; Ramage et al., 2017; Lewkowicz and Way, 2019).
RTS formation can also be initiated due to inland geomorphological triggers, such as
-
the development of thermo-erosional gullies downwards (Czudek and Demek, 1970; Jones et al., 2019),
-
mechanical riverbank and lakeshore erosion (Burn, 2000; Burn and Lewkowicz, 1990; Lewkowicz and Way, 2019; Jones et al., 2019),
-
thermokarst subsidence (Romanovskii, 1993; Voskresenskii, 2001),
-
active layer detachment slides (Lewkowicz and Harris, 2005; Lacelle et al., 2010; Swanson, 2021; Jorgenson and Osterkamp, 2005; Lewkowicz, 2007; Lamoureux and Lafrenière, 2009; Lewkowicz and Way, 2019; Jones et al., 2019), and
-
ice wedge melt that leads to the degradation of ice-rich slopes (Fraser et al., 2018).
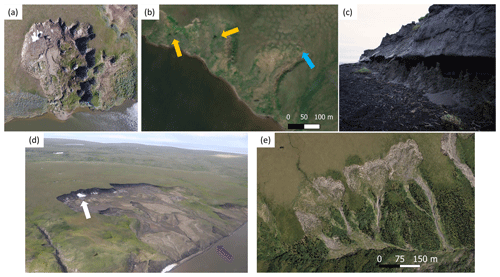
Figure 2Concurrent processes affecting RTSs. (a) Ice wedge degradation in an RTS on the Yamal Peninsula, West Siberia, Russia, in July 2018. Uncrewed aerial vehicle (UAV) photo: Artem Khomutov. (b) Thermokarst subsidence indicated by yellow arrows and ice wedge degradation indicated by a light-blue arrow in a stabilized RTS on the Gydan Peninsula, West Siberia, Russia, in July 2019. Esri basemap, GeoEye-1 satellite image. (c) Erosional niche formed due to the coastal erosion affecting an RTS in Oyagos Yar, NE Siberia, Russia, in September 2002. Photo: Guido Grosse. (d) The white arrow indicates snow packs staying over summer, and the purple arrow indicates an area where coastal erosion undercuts the coast and washes away the debris tongue of an RTS on Herschel Island, northern Canada, in July 2022. Photo from helicopter: Saskia Eppinger. (e) Active thermal erosion in RTSs that occurred within gullies near Willow River, NW Canada, in July 2023. Aerial camera image: AWI. The Esri basemap used in panel (b) has the following credits: Esri, DigitalGlobe, GeoEye, i-cubed, USDA FSA, USGS, AEX, Getmapping, AeroGRID, IGN, IGP, swisstopo, and the GIS User Community.
Two additional interesting but maybe highly site-specific inland geomorphological triggers were reported to be possible reasons for RTS reinitiation:
-
the growth of a talik that occurs in ice-rich permafrost leading to thaw subsidence and stimulating further RTS reoccurrence (Kokelj et al., 2009), and
-
the growth of a debris tongue (thawed sediments in the shape of a tongue; for details, see Sect. 3.1.8) eventually obstructing a stream valley and leading to a rise in the stream base level and further thermo-erosion that can erode and expose the ground ice and secondary RTS occurrence (Kokelj et al., 2015).
2.5 Polycyclicity
RTSs can develop in a polycyclic fashion, which means they can be active, then temporarily stabilize, and then reactivate (Mackay, 1966; Kerfoot, 1969; Kokelj et al., 2009). However, some may end off in one cycle. RTSs can be regarded as active when there is an ongoing ablation of the exposed ice and thawed material is transferred downslope. Some studies reported continued headwall retreat and thawed sediment fluxes even in slumps where the ice was covered by the sediments (Kokelj et al., 2015; Zwieback et al., 2020). The reasons for these sediment-covered slumps to retain activity were heavy rainfalls and unsuppressed heat flux to the ice.
RTSs can stabilize mostly for two reasons: (1) exposed ground ice has completely melted or (2) the exposed ice is re-buried by sediments and thermally fully insulated from further melting (Burn and Friele, 1989). Once an RTS is stabilized, pioneer vegetation starts to grow in the slump floor. Vegetation in stabilized RTSs can go through several stages of succession, and, for stabilized RTSs in Yukon, Canada, it was reported that forest and tundra communities were re-established after 35–50 years (Burn and Friele, 1989). Some researchers found that RTSs can be stabilized for up to several hundred years in West Siberia, Russia, (Leibman et al., 2014). Such long-term stabilized RTSs are labeled in some studies as ancient (Nesterova et al., 2023).
New active RTSs can form within the outline of another stabilized RTS; moreover, neighboring RTSs can grow and coalesce at some point (Lantuit and Pollard, 2008). This leads to the very complex spatial organization of nested and amalgamated RTSs of sometimes different ages. It raises additional challenges when delineating and mapping RTSs and their characteristics (van der Sluijs et al., 2023; Leibman et al., 2023a).
2.6 Concurrent processes
While triggering processes (described in Sect. 3.2) take place before RTS initiation, concurrent processes start simultaneously or soon after RTS initiation and are often further reinforced by RTS growth. Depending on the terrain, concurrent processes can have different impacts on RTSs.
If RTS initiation occurs along the ice wedge polygonal network, this also affects its further development (Fraser and Burn, 1997; Fraser et al., 2018). Ice wedge degradation can result in the rugged outline of the headwall following the morphology of wedges (Fig. 2a and b).
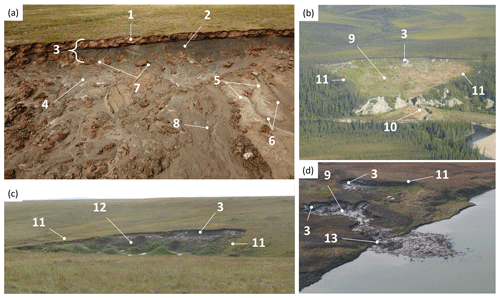
Figure 3Morphologic parts of active RTSs. (a) Yamal Peninsula, West Siberia, Russia, in July 2019. UAV photo: Nina Nesterova. (b) Alaska, USA, in August 2016. Photo from airplane: Ingmar Nitze. (c) Bykovsky Peninsula, NE Siberia, Russia, in August 2015. Field photo: Alexandra Veremeeva. (d) Gydan Peninsula, West Siberia, Russia, in 2020. Photo from helicopter: Elena Babkina. The numbers on the photos stand for the following morphologic parts. 1: headwall, i.e., the upper vertical part of the wall only; 2: headscarp; 3: headwall (or a backwall), more generally describing the entire steep wall; 4: mud pool; 5: mud levees; 6: mud gullies; 7: slump block; 8: mudflow; 9: slump floor or scar; 10: evacuation channel; 11: sidewall; 12: baydzherakhs; 13: debris tongue.
RTSs on the sea coast or near any large water bodies (lakes and rivers) where wave action takes place are affected by coastal erosion at the bluff base. This process manifests itself in washing away the debris tongue of the RTS, thus promoting further RTS growth by removing debris blockages (Burn and Friele, 1989; Are, 1998), steepening the erosional base by coastal retreat, and, in some cases, also undercutting the coast and niche-formation, adding to the further collapse of steep shore bluffs (Fig. 2e).
As mentioned above, RTSs can form due to massive ground ice exposure in thermo-erosional gullies. Usually, in such RTSs, lateral thermo-erosion continues to act simultaneously with ice ablation and thaw-related mass wasting. Sometimes lateral thermo-erosion can appear in an already existing RTS (Kerfoot, 1969; Lantuit and Pollard, 2005; Gubarkov et al., 2009). In both cases, the RTS has or develops a specific gully-like shape (Fig. 2c).
Due to specific RTS geometries and climatic conditions, thick snow packs accumulating from the wind drift of snow in the winter can remain within some RTSs over the summer (Fig. 2d). This can affect RTS development by thermal insulation (Zwieback et al., 2018). It was reported that snow packs prevented fast headwall retreat rates compared to the headwalls not covered by snow (Lacelle et al., 2015).
Thermokarst subsidence and ponding can also occur within a slump floor (Fig. 2b). This happens if the ground ice lies deeper than the level of the slump floor and if there are conditions for water to accumulate in local concavities (Leibman and Kizyakov, 2007). Meltwater streams can also go into ice wedge tunnels, disappear into sinkholes on the slump floor, and reappear further down on the slump floor.
3.1 Morphologic parts
RTSs have various morphologic parts, of which some are characteristic of all RTSs but some may be present only in certain RTS types and depend on local geological conditions (Fig. 3). Moreover, some of these RTS features can still be visible even after the RTS stabilized. Some studies use various terms to describe the same parts of RTSs, which are then synonymous terms, while other studies use the same terms but actually describe partially or fully different parts of the RTS with them. This can cause confusion when comparing RTS characteristics across different studies (Table 1).
3.1.1 Headwall and sidewalls
The term headwall is used in the literature in two ways: (1) as a broad general term for the steep wall of RTSs, where the ice is exposed (Kerfoot, 1969; Egginton, 1976; Burn and Friele, 1989; Burn and Lewkowicz, 1990; Barry, 1992), and (2) as a term for only the upper vertical part of the wall that consists of the active layer and ice-poor organic or mineral sediments (Lantuit and Pollard, 2005; Lewkowicz and Way, 2019). The second, lower part of the RTS wall, according to these authors, is a steep (20–50°) headscarp that consists of exposed ice-rich sediment or massive ground ice. Exposed ice is not only called a headscarp in the literature but sometimes also an ice face, and, in such cases, the ice face is a part of the headwall that represents the whole RTS wall in a general way (Kerfoot, 1969; De Krom, 1990; Burn and Lewkowicz, 1990; Barry, 1992).
There are several terms in the literature that are used to describe the whole RTS wall (headwall in a general way); for example, slump face (Huang et al., 2022), scarp (Mackay, 1966; Kerfoot, 1969; Egginton, 1976; Fortier et al., 2007; Wang et al., 2009; Nicu et al., 2021), and escarpment (Swanson and Nolan, 2018; Swanson, 2021). Another similar term is a backwall, and it is used to describe the whole RTS wall but separate it by its location on the back of the RTS (Lamothe and St-Onge, 1961; Worsley, 1999; Leibman et al., 2008). Those RTS walls that are located at the sides are sometimes called sidewalls (Lewkowicz, 1987b). Sidewalls can be called an optional morphologic part, since they mostly occur only in bowl-shaped morphologies.
Since a headwall is a wall with exposed ablating ice and frozen sediments, it can only be found in an active RTS. The remnants of the headwall in stabilized RTSs are sometimes called stable headwalls (Kokelj et al., 2009) or old headscarps (Zwieback et al., 2018).
3.1.2 Slump floor or scar
As a headwall retreats, it leaves a low-angle surface that can also be described as the bottom of the RTS hollow. This surface is termed a slump floor (Mackay, 1966; Lewkowicz, 1987a; Burn and Friele, 1989; De Krom, 1990; Barry, 1992; Lantuit and Pollard, 2005; Lacelle et al., 2010), highlighting its flatness, or sometimes a scar (De Krom, 1990; Barry, 1992; Kokelj et al., 2002, 2009), which originates from landslide terminology and means the bare surface that is left after the removal of the mobilized sediments by mass movement. Both of the terms are equally popular in the literature and are sometimes used interchangeably in the same study (De Krom, 1990; Barry, 1992). A slump floor or scar can be found in both active and stabilized RTSs.
3.1.3 Mud pool and mudflows
The area of the mud on the slump floor right next to the headwall is often (but not always) the place where meltwater accumulates. Some authors call this area of the RTS slump floor a mud pool (De Krom and Pollard, 1989; Lantuit and Pollard, 2005). Thawed sediments after their first accumulation in the mud pool are transported downslope by streams of meltwater. These flows of meltwater-saturated mud, depending on the amount of water, are generally called mudflows (Lamothe and St-Onge, 1961; Egginton, 1976; Lewkowicz, 1987a), earthflows/mudflows (Leibman et al., 2014), and debris flows (Murton, 2001; Lipovsky and Huscroft, 2006).
3.1.4 Mud gullies and levees
Meltwater streams can lead to the formation of mud gullies within a slump floor – erosional channels that are carved by meltwater streams into debris (Lantuit and Pollard, 2005). If transported debris stagnates and dries out, it may form mud levees bordering mudflows (Kerfoot, 1969; Lantuit and Pollard, 2005).
3.1.5 Slump block
The pieces of ice-poor, often organic-rich peaty soil covered with vegetation that slide down the headwall into the slump floor and stay rigid when moving downslope with mudflows are called slump blocks in some studies (Swanson and Hill, 2012; Kokelj et al., 2015). If these features consist of active layer soil, they generally preserve the initial undisturbed tundra vegetation. Some authors also called these blocks remnant islands (Burn and Friele, 1989; Bartleman et al., 2001).
3.1.6 Baydzherakh(s)
Baydzherakhs (from the Yakutian language, but now a more commonly accepted term) are conical mounds in the slump floor of RTSs representing largely still-frozen remnants of ice wedge polygon centers where the surrounding large polygonal ice wedges have already thawed substantially. They are typical of RTSs located on upland slopes with ice-rich deposits and large polygonal ice wedges up to 50 m thick (e.g., the Yedoma Ice Complex) (Tikhomirov, 1958; Czudek and Demek, 1970; Zhigarev, 1975; Pizhankova, 2011; Séjourné et al., 2015). Baydzherakhs can reach significant sizes: up to 11 m in height, 15 m in width, and 20 m in length (Tikhomirov, 1958). Thus, they can be found not only in active but also in stabilized RTSs. As a typical feature of Yedoma upland slopes, baydzherakhs are widely distributed in the Yedoma Ice Complex regions of eastern and northeastern Siberia, Alaska, and northwestern Canada (Strauss et al., 2021), as well as in other areas formed by ice-rich deposits with large polygonal ice wedges. Baydzherakhs will therefore not form in areas where RTSs are formed in deposits with thick ice layers.
3.1.7 Evacuation channel
Depending on the morphology of an RTS, thawed sediments and meltwater (debris) can leave the slump floor through the trench connecting the slump floor and the base level. This optional morphologic part of RTSs is termed an evacuation channel (Lacelle et al., 2004, 2010; Delaney, 2015).
3.1.8 Debris tongue
Thawed sediments and meltwater (debris) moving downslope can eventually escape from the slump floor directly or via an evacuation channel. Once this happens, thawed sediments accumulate in the shape of a “tongue” on any surface where an RTS outflow ends. Such features are generally called debris tongues (Worsley, 1999; Kokelj et al., 2015; Segal et al., 2016) but are sometimes referred to as mud or slump lobes (Lantuit and Pollard, 2005).
3.1.9 Edge and dropwall
The term edge of an RTS is used in the literature to indicate (1) the outline of the whole feature (van der Sluijs et al., 2023) and (2) the boundary line of active retreat (Cassidy et al., 2017; Leibman et al., 2021, 2023; Kizyakov et al., 2023). There is also the term outline itself that is used to describe the whole area of the RTS landform (Burn, 2000) or only the polygon that is regarded as the RTS detected by automated mapping methods (Yang et al., 2023). Furthermore, the edge of the RTS is also sometimes classified into the upper edge, meaning the boundary line of active retreat of the headwall (Kizyakov et al., 2023), and the lower edge, meaning the boundary line of the cliff retreat for RTSs on sea coasts (Leibman et al., 2008, 2021). The face (cliff) from the lower edge of coastal RTSs to the beach level has been called a dropwall (Leibman et al., 2021) to differentiate this morphologic part of the RTS from the rest of the coastal cliff.
3.2 Landforms
3.2.1 Retrogressive thaw slump (RTS)
According to the International Permafrost Association Multi-Language Glossary of Permafrost and Related Ground-Ice Terms (van Everdingen, 2005), an RTS is defined as “a slope failure resulting from thawing of ice-rich permafrost. Retrogressive thaw slumps consist of a steep headwall that retreats in a retrogressive fashion due to thawing and a debris flow formed by the mixture of thawed sediment and meltwater that slides down the face of the headwall and flows away. Such slumps are common in ice-rich glaciolacustrine sediments and fine-grained diamictons.”
3.2.2 Cryogenic earthflow
In Russian literature, the word cryogenic is usually used to describe the periglacial nature of the processes. It refers to thermophysical, physicochemical, and physicomechanical processes occurring in freezing, frozen, and thawing deposits (van Everdingen, 2005). This term is usually omitted in the literature in English (Poppe and Brown, 1976).
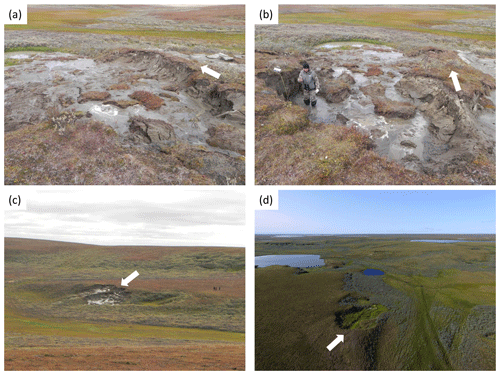
Figure 4Examples of two active cryogenic earthflows next to each other. (a–c) Photos in central Yamal, West Siberia, Russia, taken on a photo camera in 2012 and stabilized. (d) A photo taken from a UAV in 2017. The arrow indicates the direction of flow. Photos: Artem Khomutov.
The term cryogenic earthflow was introduced by Leibman (1997), meaning a viscous or viscoelastic flow of water-saturated soil of the active layer sliding on the surface of massive ground ice bodies or the table of ice-rich permafrost. Examples of cryogenic earthflows in central Yamal are demonstrated in Fig. 4.
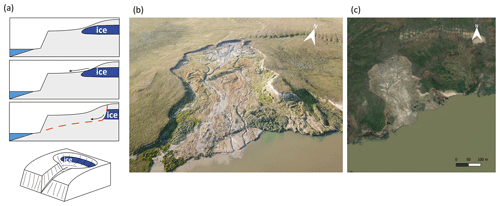
Figure 5(a) Scheme of thermocirque formation. The black arrow indicates the direction of mass movement of thawed material, and the dashed red line stands for the cross-section of the headwall and the slump floor (see Sect. 3.1.2). Note that the scheme demonstrates the particular ice morphology of a layer of tabular massive ground ice (adapted from Kizyakov, 2005) but may also consist of other forms of ice-rich ground. An example of a thermocirque in central Yamal, West Siberia, Russia, is shown in panel (b) in a UAV photo taken in August 2019 (photo: Artem Khomutov) and (c) in a WorldView-2 satellite image from July 2018 (Source: Esri satellite basemap). The Esri basemap used in panel (c) has the following credits: Esri, DigitalGlobe, GeoEye, i-cubed, USDA FSA, USGS, AEX, Getmapping, AeroGRID, IGN, IGP, swisstopo, and the GIS User Community.
3.2.3 Thermocirque
The term thermocirque was first mentioned by Czudek and Demek (1970, in English) to describe “amphitheatrical hollows” that occur after ice wedge melt in the gullies on the river banks in Yakutia (Russia). Thermocirques, according to the authors, have “a vertical and overhanging slope at the head and an uneven floor”. In the Russian-language literature, the term thermocirque was sometimes used interchangeably with the term thermokar when describing a round or cirque-like hollow on the river banks or lake shores composed of icy permafrost (Grigoriev and Karpov, 1982, in Russian; Voskresenskii, 2001, in Russian). Following the development of theoretical concepts of cryogenic landsliding (Sects. 3.2.3 and 3.2.4), the term thermocirque was defined as an extensive landform resulting from a series of multi-aged cryogenic earthflows (Leibman, 2005; Leibman et al., 2014). The scheme visualizing thermocirque formation and an example of a thermocirque in central Yamal, Russia, are demonstrated in Fig. 5.
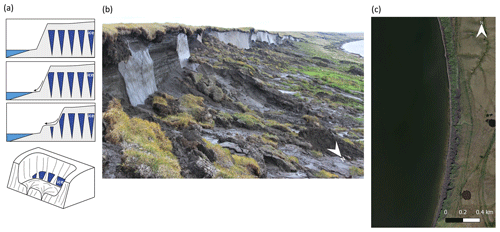
Figure 6(a) Scheme of thermoterrace formation. The black arrow indicates the direction of mass movement of thawed material; note that the scheme demonstrates the particular ground ice morphology of a layer with large ice wedges (adapted from Kizyakov, 2005) but may also consist of other ground ice morphologies. An example of thermoterraces on the Bykovsky Peninsula, NE Siberia, Russia, is shown in panel (b) in a photo taken on the ground in August 2016 (photo: Alexander Kizyakov) and (c) in a WorldView-2 satellite image from August 2020 (Esri satellite basemap). The Esri basemap used in panel (c) has the following credits: Esri, DigitalGlobe, GeoEye, i-cubed, USDA FSA, USGS, AEX, Getmapping, AeroGRID, IGN, IGP, swisstopo, and the GIS User Community.
3.2.4 Thermoterrace
The term thermoterrace was first mentioned by Ermolaev (1932, in Russian) to describe “picturesque outcrops of ice falling vertically onto a narrow, 1–2 m wide space located along the seashore along the edge of the ice wall that can reach 30–35 m”. The local term to describe these icy cliffs was muus kygams, or in the Yakutian language (Ermolaev, 1932). The more precise definition of thermoterrace was given by Zenkovich and Popov (1980) as a terrace-like area in the upper part of the icy cliff at the seashore that results from the cliff retreat due to the thermal influence of warm air and solar radiation. Thermoterraces were reported to reach up to a few kilometers in length along the coast and more than 200 m in width (Are et al., 2005). A scheme visualizing thermoterrace formation based on Kizyakov (2005) and an example of a thermoterrace on the Bykovsky Peninsula, Yakutia, Russia, are shown in Fig. 6.
3.2.5 Active layer detachment slide
Another closely related slope landform linked to RTS formation (see Sect. 3.2) is an active layer detachment (ALD) slide or failure. The term ALD is prevalent in recent publications (Blais-Stevens et al., 2015; Balser, 2015), yet, unlike RTS, there is no universally endorsed term in the Glossary to describe ALD phenomena (van Everdingen, 2005):
-
Active layer failure. “A general term referring to several forms of slope failures or failure mechanisms commonly occurring in the active layer overlying permafrost” (synonym not recommended: skin flow),
-
Detachment failure. “A slope failure in which the thawed or thawing portion of the active layer detaches from the underlying frozen material” (synonyms not recommended: skin flow, active layer glide).
French (2018) defines active layer detachment slides as rapid slope failures restricted to the active layer that generally occur on the middle or upper slopes. In one of several classic works on ALD, Lewkowicz (1990) defines ALD as being a rapid mass movement on permafrost slopes without strict limitation to the active layer: “failure involves the unfrozen mass detaching from the underlying substrate and sliding downslope over a thawing ice-rich zone within the active layer or the upper part of the permafrost.” Examples of mass movements that seem deeper than the active layer and are nevertheless termed ALD are shown in Rudy et al. (2016).
3.2.6 Cryogenic translational landslide
The term cryogenic translational landslide (CTL) was suggested by Kaplina (1965, in Russian), and the definition was later elaborated in further publications based on observations in central Yamal, Russia (Leibman and Egorov, 1996; Leibman, 1997; Leibman et al., 2014). The definition of CTL summarized from the abovementioned publications can be phrased as a single-time lateral displacement of a thawed soil block sliding on the surface of the seasonal ice formed at the active layer base. This type of seasonal ice is formed due to the active layer's upward freezing, ice aggradation at the base of the active layer, and later melting (Leibman et al., 2014; Lewkowicz, 1990). Examples of a CTL in central Yamal are shown in Fig. 7.
3.3 Formation process
The process of RTS formation in the recent literature is termed in two different ways: thermokarst and thermodenudation.
3.3.1 Thermokarst
The term thermokarst was first suggested by Ermolaev (1932) to describe the surface subsidence due to the melting of ground ice as a similarity to the karst process by dissolution. However, in the context of RTS formation processes, the term thermokarst is mostly referred to in the North American literature as a set of processes that lead to the occurrence of specific landforms due to the thawing of ice-rich permafrost or the melting of massive ground ice (Kokelj and Jorgenson, 2013).
3.3.2 Thermodenudation
The term thermodenudation was originally suggested by Panov (1936), defining “the influence of the sun in a direct or transformed form through soil or water on the sediments containing a certain amount of ice cement or ice masses, as well as on bedrock with negative temperature … that leads to mass-wasting as well as some forms of thermo-erosion or thermokarst”. In the context of RTS formation, this term has been used to refer to ground ice thaw and slope mass waste (Leibman et al., 2021) and to the retreat of upper bluff edges along coastal RTSs (Günther et al., 2012).
4.1 Divergent terminologies
The terminology used to describe the RTS formation processes and related landforms in 21st-century publications has historical roots in the distinctive scientific approaches developed in Russia and North America (both Canada and the USA) during the 20th century.
The process of RTS formation following the initiation by various triggers and further development into the landform has been neither named nor specifically classified in classical works on RTS and exposed ground ice (Mackay, 1966, 1970; Rampton and Mackay, 1971; Lewkowicz, 1987a; Burn and Friele, 1989).
In the literature of the 20th century, this process was often termed solifluction, thermokarst, and thermodenudation. Initially, none of these three terms took the more specific formation of RTSs into account in their definitions. At some point, however, the definitions of these three terms were expanded to include RTS formation. The process of RTS formation was also previously very broadly referred to as the process of erosion (Lamothe and St-Onge, 1961), but this term was later no longer used in publications in this context.
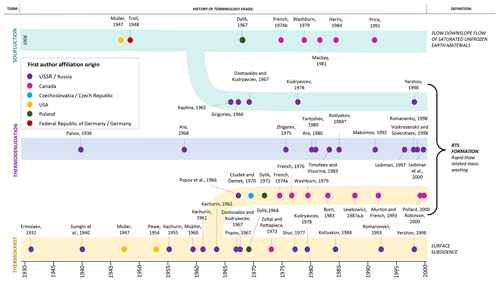
Figure 8Chronology of the usage of different RTS-related terms by a selection of the most cited authors in the 20th century. Three wide, color-coded lines represent the terms on the left-hand side, and the main process is defined on the right-hand side. The dots represent publications and are color-coded based on the origin of the first author affiliation.
The general chronology of usage of these three terms, which have different definitions in the 20th century, is shown in Fig. 8. While this chronology graph has some limitations due to (a) the ambiguity of some definitions, (b) definition reformulation by some authors through their later publications, and (c) usage of several terms for the same process, it helps us understand how the RTS terminology evolved in the scientific literature and how different schools of thought influenced its development.
The term solifluction was first introduced by Anderson (1906) and describes the process of slow downslope movement of saturated unfrozen materials (van Everdingen, 2005). In the non-Russian-language literature, this term has always been used for very slow movements up to several centimeters per year (Smith, 1988) and never for the rapid mass wasting that can lead to RTSs. Meanwhile, Russian-language authors have included the process of slumping into the solifluction, calling it rapid solifluction. Probably the most remarkable publication with such a statement was issued by Kaplina (1965). The concept of rapid solifluction was later criticized by Dylik (1967) and Leibman (1997) for summarizing processes that have process rates differing by several orders of magnitude under one term. Nevertheless, this approach of referring to the RTS formation process as rapid solifluction was frequently used in the literature until the end of the 20th century. The last publication in which rapid solifluction was mentioned in connection with the formation of RTS was by Yershov (1998).
In general, the term thermokarst has mostly been used by Russian-language researchers to describe the subsidence of the land surface (Sumgin et al., 1940; Kachurin, 1955; Mukhin, 1960; Dostovalov and Kudryavcev, 1967; Shur, 1977; Romanovskii, 1993; and many more later). Some exceptions can be found in two publications of Popov: one in English (Popov et al., 1966), where he included the slumping process in thermokarst, and another one in French (Popov, 1956), where his definition of thermokarst was not purely limited to the process of subsidence. Meanwhile, a different approach was suggested by Czudek and Demek (1970), who put the RTS formation process under the umbrella of the thermokarst term. They proposed two types of thermokarst: down-wearing, which included only subsidence, and back-wearing, which included the RTS formation. This approach was supported by French (1976), who extended this term by adding thermal erosion to it. The definition by French (1976) of thermal erosion as “a dynamic process `wearing away' by thermal means, i.e., melting of ice” differs from the one in the Glossary, where the main erosional agent is moving water: “the erosion of ice-rich permafrost by the combined thermal and mechanical action of moving water.” This is the reason why the RTS formation process is sometimes called thermal erosion. For example, Burn (1983) relates the process of RTS formation to thermal erosion, which he in turn describes as part of the thermokarst process.
Since French (1976) expanded the definition of thermokarst processes to encompass slope processes and, in particular, thaw slumping, the RTS formation process has consistently been perceived as a thermokarst process in the North American literature (Washburn, 1979; Burn, 1983) or is sometimes specified as hillslope thermokarst (Gooseff et al., 2009). There was no agreement among scholars on the terminology of RTS itself. RTSs were termed in the literature as tundra mudflows (Lamothe and St-Onge, 1961), ground ice slumps (Mackay, 1966; French, 1976), retrogressive thaw flow slides (Hughues, 1972), bi-modal flows (McRoberts and Morgenstern, 1974), or just thaw slumps (Washburn, 1979). The 1998 Glossary (van Everdingen, 2005) initially recommended using the term “retrogressive thaw slump”, though alternative terms persist in later literature, such as “retrogressive thaw flowslides (thawslides)” (Wolfe et al., 2001) and “retrogressive thaw flows” (Highland and Bobrowsky, 2008).
Unlike RTS, the process of ALD was not always classified as thermokarst in the North American literature (Lewkowicz, 1990; Lewkowicz and Harris, 2005, etc.). For example, French (1976, 2018) describes ALD under the section of “Rapid mass movements” but not “Thermokarst” in all editions of his textbook The Periglacial Environment. ALDs are included in the list of thermokarst processes described for Alaska by Jorgenson et al. (2008) and are classified as hillslope thermokarst by Gooseff et al. (2009). Recent publications tend to include the process of ALD in the concept of thermokarst (Kokelj and Jorgenson, 2013; Ramage et al., 2019; Kokelj et al., 2023).
Additional definitions of thaw-related slope processes in the North American literature worthy of mention can be found in The Landslide Handbook – A Guide to Understanding Landslides of the United States Geological Survey by Highland and Bobrowsky (2008). In the section “Flows in permafrost”, the authors define ALD as the “rapid flow of shallow layer of saturated soil and vegetation” that moves over but on the underlying permafrost. Retrogressive thaw flows (as an analog term for RTSs) are described as the features resulting from the thawing of exposed buried ice lenses (Highland and Bobrowsky, 2008).
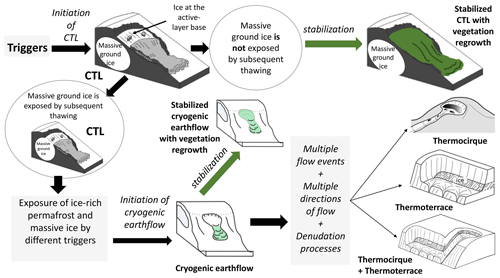
Figure 9Conceptual diagram of the interrelation of different terms in the Russian literature used to describe the RTS formation process and the resulting landforms. CTL stands for cryogenic translational landslide. Note that what is shown as massive ground ice in the diagram may have different characteristics in different regions and could include buried glacial ice, thick ice layers, or large syngenetic ice wedges.
The term thermodenudation (sometimes also thermal denudation) has never been properly introduced in the English-language literature; however, it is widely used in the Russian-language permafrost literature with two types of definitions: narrow and wide. For the initial (narrow) definition, see Sect. 3.3.2. Are (1968) used this term to describe the thermal effect of solar radiation and sensible heat affecting the retreating ice-rich coastal cliffs. Zhigarev (1975) highlighted the importance of the slope in his definition of thermodenudation as “a complex of gravitational and erosive processes that develop on slopes during thawing of ice-rich deposits of various genesis”. The only wide definition of thermodenudation was introduced in the Glossary of Glaciology (Kotlyakov, 1984) as “a set of cryogenic destructive processes and the transfer of the products of destruction downwards. Thermodenudation includes cryogenic weathering, nivation, cryogenic slope processes (mass movements), thermal erosion, thermal coastal erosion, thermokarst, and thermal suffosion”. This wide definition by Kotlyakov (1984) of a thaw-related process is quite similar to the expanded version of the thermokarst term by French (1976).
In the context of RTS formation, the term thermodenudation was widely applied in its narrow definition as a set of slope processes associated with the thawing of ice-rich deposits and leading to the occurrence of mass movements and concavities of different shapes (Fartyshev, 1980; Romanenko, 1998; Leibman et al., 2021; and many more). These mass movements were classified by Leibman (1997) into two types depending on the sliding surface: cryogenic translational landslides on the seasonal ice in the base of the active layer (for a detailed definition, see Sect. 3.2.3) and cryogenic earthflows on the massive ice or icy permafrost (for a detailed definition, see Sect. 3.2.4).
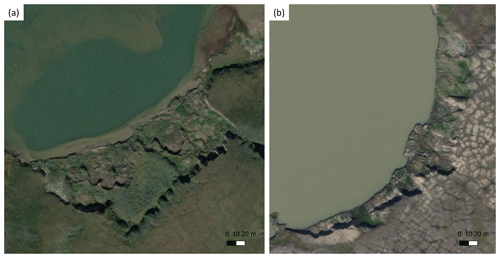
Figure 10Examples of combined RTS morphologies with thermoterraces and thermocirques. (a) Thermocirque(s) growing into a stabilized thermoterrace in central Yamal, West Siberia, Russia as seen in a WorldView-3 satellite image from August 2018 (Esri satellite basemap). (b) Thermocirque and thermoterrace merging at the coast into one outline in western Yamal, West Siberia, Russia as seen in a WorldView-3 satellite image from August 2018 (Esri satellite basemap). The Esri basemap has the following credits: Esri, DigitalGlobe, GeoEye, i-cubed, USDA FSA, USGS, AEX, Getmapping, AeroGRID, IGN, IGP, swisstopo, and the GIS User Community.
Figure 9 demonstrates a conceptual scheme that explains the interrelation of different processes and lists the landforms resulting from thermodenudation (in a narrow definition) in the Russian literature. When the cryogenic translational landslides do not lead to the exposure of ice-rich permafrost or massive ground ice, the surface of exposed bare soil gets revegetated (Khomutov and Leibman, 2016). Otherwise, if the icy deposits or the massive ice body are exposed because of this disturbance, a cryogenic earthflow can occur. Such features can also stabilize if the accumulation of drying sediments insulates the exposed ice. Once further thawing is suspended, the surfaces of these landforms get revegetated (Fig. 9) (Leibman, 2005). In contrast, the continued expansion of the flow and mass movements in several directions involving additional cryogenic processes leads to the formation of mature landforms defined in the literature as thermocirques (for a detailed definition, see Sect. 3.2.5) and thermoterraces (for a detailed definition, see Sect. 3.2.6). Thermocirques are reported in the literature to exhibit amphitheater-like shapes (Leibman et al., 2014), while thermoterraces are described as landforms elongated along the coast or the shore with coastal erosion contributing to the cut of their lower part (Are et al., 2005). The combinations of these two landforms are also observed in some regions (Leibman et al., 2023b) (Fig. 10). This is usually found in two settings: one or more thermocirques form and grow in a former but now stabilized thermoterrace (Fig. 10a), or an originally separate thermocirque and thermoterrace merge at the coast into one outline (Fig. 10b).
4.2 Overlap in terminology
The terms described above refer to similar phenomena of the RTS formation process and resulting landforms, leading to inevitable similarities and overlaps but also differences (Table 2).
The term cryogenic translational landslide corresponds to shallow active layer detachment slide in the North American literature that is triggered by high pore-water pressure and low effective strength (Lewkowicz, 2007). Cryogenic earthflow corresponds to a deep ALD in the North American literature and is the very early stage of RTS formation. Thermocirque and thermoterrace signify the mature stage of RTSs of different morphology.
The process of RTS formation can generally be described as a mass-wasting (landsliding) process resulting from the melting of massive ground ice exposed due to various triggers. Regardless of which terminology is used (thermodenudation in the Russian literature or thermokarst in the North American literature), it can be seen as a sequence of physical events (Fig. 11): trigger, massive ground ice exposure, ice ablation, thaw-related mass movement, and landform formation. The first two physical events (trigger + massive ground ice exposure) are usually regarded as the RTS initiation stage. Triggers of massive ice exposure lead to mass wasting and RTS landform occurrence.
4.3 Limitations of divergent terminology
All the terms used to explain RTS formation in both the North American and Russian literature have their limitations. The usage of a single term, thermokarst, for a wide variety of processes leads to confusion about the direction of the physical process happening: whether it is the vertical lowering of the surface in the case of thermokarst lakes or lateral mass movement in the case of RTS occurrence. Since the term thermokarst in this case incorporates both directions of the process, it is crucial to clearly state that RTS formation implies a back-wearing thermokarst (or hillslope thermokarst). More confusion can appear when talking about mass movements that are deeper than the active layer and slide on the surface of massive ground ice. In the North American literature, such landslides can still be called active layer detachment slides. However, since these mass movements expose massive ground ice, the retrogression can already start, which means that it is already an early-stage RTS.
Such mass movements on the surface of massive ground ice are called cryogenic earthflows and are regarded as early-stage RTSs in the Russian literature. However, it is difficult to distinguish an early-stage RTS (cryogenic earthflow) from a mature-stage RTS (thermocirque), since mature RTSs can also be of small sizes. Clear separation of these two categories is almost impossible with remote-sensing data and is quite demanding in the field, since it requires thorough knowledge of the environment and the dynamics of each RTS.
The definitions of thermocirque and thermoterrace presented in the literature are based on the morphology of the features. Considering morphology as a distinguishing factor can be subjective, since no established curvature values exist in the literature to differentiate them. In some cases, a thermoterrace can appear more curved, rather resembling a thermocirque. In contrast, a thermocirque can further elongate in width following the initial shape of massive ground ice (e.g., Fig. 1 in Swanson and Nolan, 2018), while its mudflow can reach the neighboring water body base level.
4.4 RTS definition in the Glossary
With a large number of recent RTS mapping studies in different permafrost regions, it has become clear that RTS characteristics and morphologies vary widely, that RTSs can occur in a range of different permafrost and ground ice settings, and that they feature processes important for understanding their dynamics and environmental impacts. However, these aspects are not yet covered by the current definition of a retrogressive thaw slump in the International Permafrost Association Multi-Language Glossary of Permafrost and Related Ground-Ice Terms (van Everdingen, 2005) (see Sect. 3.2.1). This definition is rather short and describes a portion of RTS characteristics: it is limited in its scope and does not capture the full breadth of RTS variability emerging from the many studies. In particular, the definition only focuses on the active stage of RTSs, while the polycyclic nature of many RTSs also includes the stages of stabilization without activity. Moreover, this definition does not reflect the variety of possible morphologies as horseshoe-like (thermocirques) or elongated along the coast (thermoterrace) and different stages of landform evolution. Furthermore, some other settings also feature slump-like landforms that exhibit a similar headwall back-wasting but were not covered in this review. Such slumps, for example, occur on recent dead-ice moraines that experience retrogressive rotational sliding or back-slumping of the ice-cored slopes (Kjær and Krüger, 2001). Thus, a clear distinction should be made in the definition. We recommend considering these points when preparing the next International Permafrost Association Multi-Language Glossary of Permafrost and Related Ground-Ice Terms.
4.5 Missing terminology
Our review of morphologic elements of RTSs (see Sect. 3.1) showed that there is no term to describe unthawed permafrost remnants within a slump floor. The term slump block, in our opinion, fits the best to explain pieces of soil with vegetation that move downwards, while the term remnant island sounds rather confusing because it does not assume the moving nature of such a feature. We rather suggest using the term remnant island to describe unthawed permafrost remnants within a slump floor. These remnant islands are generally larger than slump blocks and do not move, since they still have unthawed cores. An example of such a remnant island is shown in Fig. 12.
Retrogressive thaw slumps are complex permafrost region landforms which, despite recent wide scientific interest, are still studied very differently in terms of terminology. Based on our review of the literature and terminology, we draw the following conclusions:
-
The RTS formation process is currently explained with two different terms (thermokarst and thermodenudation) in the North American and Russian literature based on different theoretical views that were formed in the 20th century.
-
RTS is a general umbrella term applied to different stages of landform activity and also to a variety of mass-wasting landforms on slopes with ice-rich permafrost (thermocirque/thermoterrace).
-
RTSs can differ in shape, triggers, ground ice types, position in the relief, activity, concurrent processes, and spatial organization.
-
For active RTSs we identified four essential morphologic parts (headwall, slump floor, mudflow, and edge), while nine additional parts may or may not be present in an RTS.
The study of RTS formation and accompanying processes is important to better understand how rapid mass wasting on permafrost slopes can mobilize sediment, meltwater, carbon, and nutrients; how biogeochemical dynamics are influenced by specific processes during the RTS formation and growth; and how RTSs may pose hazards to infrastructure. More clarity on the existing terminology and scientific views will foster this understanding and can guide new research.
Data and/or further information regarding the data used can be obtained from the author(s) upon request.
NN: conceptualization, resources (literature sources), investigation, and writing (original draft preparation). ML: conceptualization, supervision, and writing (review and editing). AK: supervision and writing (review and editing). HL: conceptualization, supervision, and writing (review and editing). IT: resources (literature sources) and writing (review and editing). IN: writing (review and editing). AV: writing (review and editing). GG: conceptualization, supervision, and writing (review and editing).
The contact author has declared that none of the authors has any competing interests.
Publisher's note: Copernicus Publications remains neutral with regard to jurisdictional claims made in the text, published maps, institutional affiliations, or any other geographical representation in this paper. While Copernicus Publications makes every effort to include appropriate place names, the final responsibility lies with the authors.
We would like to acknowledge and appreciate the helpful discussion on RTS terminology with Steven V. Kokelj and Jurjen van der Sluijs during an earlier stage of the paper. This review is part of the efforts of the RTSInTrain Action Group led by Anna Liljedahl on retrogressive thaw slumps by the International Permafrost Association. Marina Leibman and Ilya Tarasevich were funded by the state assignment of the Ministry of Science and Higher Education of the Russian Federation (grant no. FWRZ-2021-0012). Ingmar Nitze and Guido Grosse were supported by the Google Permafrost Discovery Gateway. Alexander Kizyakov was funded by the state assignment “Evolution of the cryosphere under climate change and anthropogenic impact” (grant no. 121051100164-0). Nina Nesterova was funded by a DAAD fellowship (grant no. 57588368).
This research has been supported by the Deutscher Akademischer Austauschdienst (grant no. 57588368).
The article processing charges for this open-access publication were covered by the Alfred-Wegener-Institut Helmholtz-Zentrum für Polar- und Meeresforschung.
This paper was edited by Christian Hauck and reviewed by two anonymous referees.
Anderson, J.: Solifluction, a component of sub-aerial denudation, J. Geol., 14, 91–112, https://doi.org/10.1086/621284, 1906.
Ardelean, F., Onaca, A., Chetan, M.-A., Dornik, A., Georgievski, G., Hagemann, S., Timofte, F., and Berzescu, O.: Assessment of Spatio-Temporal Landscape Changes from VHR Images in Three Different Permafrost Areas in the Western Russian Arctic, Remote Sens.-Basel, 12, 3999, https://doi.org/10.3390/rs12233999, 2020.
Are, F. E.: Evolution of the relief of thermal abrasion coasts, in: Geography series, Proceedings of the USSR Academy of Sciences, Nauka Publisher, Moscow, 92–100, 1968 (in Russian).
Are, F. E.: The contribution of shore thermoabrasion to the Laptev Sea sediment balance, in: Permafrost: Proceedings of the Seventh International Conference, Yellowknife, NWT, 23–27 June, Yellowknife, NWT, Canada, 25–30, 1998.
Are, F. E., Grigoriev, M. N., Hubberten, H.-W., and Rachold, V.: Using thermoterrace dimensions to calculate the coastal erosion rate, Geo-Mar. Lett., 25, 121–126, https://doi.org/10.1007/s00367-004-0193-y, 2005.
Babkina, E. A., Leibman, M. O., Dvornikov, Yu. A., Fakashchuk, N. Yu., Khairullin, R. R., and Khomutov, A. V.: Activation of Cryogenic Processes in Central Yamal as a Result of Regional and Local Change in Climate and Thermal State of Permafrost, Russ. Meteorol. Hydro.+, 44, 283–290, https://doi.org/10.3103/S1068373919040083, 2019.
Balser, A. W.: Retrogressive thaw slumps and active layer detachment slides in the Brooks Range and foothills of northern Alaska: terrain and timing, University of Alaska Fairbanks, 2015.
Balser, A. W., Jones, J. B., and Gens, R.: Timing of retrogressive thaw slump initiation in the Noatak Basin, northwest Alaska, USA, J. Geophys. Res.-Earth, 119, 1106–1120, https://doi.org/10.1002/2013JF002889, 2014.
Barry, P.: Ground ice characteristics in permafrost on the Fosheim Peninsula, Ellesmere Island, NWT: a study utilizing ground probing radar and geomorphological techniques, McGill University, 135 pp., 1992.
Bartleman, A., Miyanishi, K., Burn, C. R., and Côté, M. M.: Development of Vegetation Communities in a Retrogressive Thaw Slump near Mayo, Yukon Territory: A 10-year Assessment, ARCTIC, 54, 149–156, 2001.
Bernhard, P., Zwieback, S., Bergner, N., and Hajnsek, I.: Assessing volumetric change distributions and scaling relations of retrogressive thaw slumps across the Arctic, The Cryosphere, 16, 1–15, https://doi.org/10.5194/tc-16-1-2022, 2022.
Biskaborn, B. K., Smith, S. L., Noetzli, J., Matthes, H., Vieira, G., Streletskiy, D. A., Schoeneich, P., Romanovsky, V. E., Lewkowicz, A. G., Abramov, A., Allard, M., Boike, J., Cable, W. L., Christiansen, H. H., Delaloye, R., Diekmann, B., Drozdov, D., Etzelmüller, B., Grosse, G., Guglielmin, M., Ingeman-Nielsen, T., Isaksen, K., Ishikawa, M., Johansson, M., Johannsson, H., Joo, A., Kaverin, D., Kholodov, A., Konstantinov, P., Kröger, T., Lambiel, C., Lanckman, J.-P., Luo, D., Malkova, G., Meiklejohn, I., Moskalenko, N., Oliva, M., Phillips, M., Ramos, M., Sannel, A. B. K., Sergeev, D., Seybold, C., Skryabin, P., Vasiliev, A., Wu, Q., Yoshikawa, K., Zheleznyak, M., and Lantuit, H.: Permafrost is warming at a global scale, Nat. Commun., 10, 264, https://doi.org/10.1038/s41467-018-08240-4, 2019.
Blais-Stevens, A., Kremer, M., Bonnaventure, P. P., Smith, S. L., Lipovsky, P., and Lewkowicz, A. G.: Active Layer Detachment Slides and Retrogressive Thaw Slumps Susceptibility Mapping for Current and Future Permafrost Distribution, Yukon Alaska Highway Corridor, in: Engineering Geology for Society and Territory – Volume 1, edited by: Lolliino, G., Manconi, A., Clague, J., Shan, W., and Chiarle, M., Springer International Publishing, Cham, https://doi.org/10.1007/978-3-319-09300-0_86, 449–453, 2015.
Burn, C. R.: Investigations of thermokarst development and climatic change in the Yukon Territory, Carleton University, Ottawa, Ontario, Canada, 142 pp., 1983.
Burn, C. R.: The thermal regime of a retrogressive thaw slump near Mayo, Yukon Territory, Can. J. Earth Sci., 37, 967–981, 2000.
Burn, C. R. and Friele, P. A.: Geomorphology, Vegetation Succession, Soil Characteristics and Permafrost in Retrogressive Thaw Slumps near Mayo, Yukon Territory, ARCTIC, 42, 31–40, https://doi.org/10.14430/arctic1637, 1989.
Burn, C. R. and Lewkowicz, A. G.: Canadian landform examples-17 retrogressive thaw slumps, Can. Geogr./Geogr. Can., 34, 273–276, 1990.
Cassidy, A. E., Christen, A., and Henry, G. H. R.: Impacts of active retrogressive thaw slumps on vegetation, soil, and net ecosystem exchange of carbon dioxide in the Canadian High Arctic, Arctic Science, 3, 179–202, https://doi.org/10.1139/as-2016-0034, 2017.
Clark, A., Moorman, B., Whalen, D., and Fraser, P.: Arctic coastal erosion: UAV-SfM data collection strategies for planimetric and volumetric measurements, Arctic Science, 7, 605–633, https://doi.org/10.1139/as-2020-0021, 2021.
Costard, F., Dupeyrat, L., Séjourné, A., Bouchard, F., Fedorov, A., and Saint-Bézar, B.: Retrogressive Thaw Slumps on Ice-Rich Permafrost Under Degradation: Results From a Large-Scale Laboratory Simulation, Geophys. Res. Lett., 48, e2020GL091070, https://doi.org/10.1029/2020GL091070, 2021.
Czudek, T. and Demek, J.: Thermokarst in Siberia and Its Influence on the Development of Lowland Relief, Quaternary Res., 1, 103–120, https://doi.org/10.1016/0033-5894(70)90013-X, 1970.
Dall, W. H.: Notes on Alaska and the vicinity of Bering Strait, Am. J. Sei., 121, 104–111, 1881.
Dallimore, S. R., Wolfe, S. A., and Solomon, S. M.: Influence of ground ice and permafrost on coastal evolution, Richards Island, Beaufort Sea coast, N. W. T., Can. J. Earth Sci., 33, 664–675, https://doi.org/10.1139/e96-050, 1996.
De Krom, V.: A geomorphic investigation of retrogressive thaw slumps and active layer slides on Herschel Island, Yukon Territory, PhD Thesis, McGill University, Montreal, Canada, 1990.
De Krom, V. and Pollard, W. H.: The occurrence of ground ice slumps on Herschel Island, Yukon Territory, Musk-Ox, 37, 1–7, 1989.
Delaney, S.: Impacts of retrogressive permafrost thaw slumps on aquatic systems in the Peel Plateau, Northwest Territories, Canada, PhD Thesis, Carleton University, 2015.
Dostovalov, B. N. and Kudryavcev, V. A.: General permafrost science, Moscow State University, Moscow, Russia, 403 pp., 1967 (in Russian).
Dylik, J.: Solifluxion, Congelifluxion and Related Slope Processes, Geogr. Ann. A, 49, 167–177, https://doi.org/10.1080/04353676.1967.11879747, 1967.
Dylik, J.: Thermokarst, The Encyclopedia of Geomorphology, Encyclopedia of Earth Sciences Series, Volume III, edited by: Fairbridge, R. W., Reinhold Book Corporation, New York, Amsterdam, London, 1149–1151, 1968.
Dylik, J.: Current thermal erosion and its frozen traces in the Polish landscape, B. Acad. Pol. Sci., XIX, 1149–1151, 1971 (in French).
Egginton, P.: Thermokarst and related geomorphic processes, eastern Banks Island, NWT, PhD Thesis, University of Ottawa, Ottawa, Canada, 1976.
Ermolaev, M.: Geologic and Geomorphologic Essay on the Bol'shoi Lyakhov Island, Polar Geophysical Station on Bol'shoi Lyakhov Island. Part I. Organization and Work of the Station in 1927–1930, 55–61, 147–228, 1932 (in Russian).
Fartyshev, A. I.: Thermodenudational landforms in the north of Yakutia, in: Permafrost studies in developed areas of the USSR, Nauka, Novosibirsk, USSR, 128–132, 1980 (in Russian).
Fortier, D., Allard, M., and Shur, Y.: Observation of rapid drainage system development by thermal erosion of ice wedges on Bylot Island, Canadian Arctic Archipelago, Permafrost Periglac., 18, 229–243, https://doi.org/10.1002/ppp.595, 2007.
Fraser, R., Kokelj, S., Lantz, T., McFarlane-Winchester, M., Olthof, I., and Lacelle, D.: Climate Sensitivity of High Arctic Permafrost Terrain Demonstrated by Widespread Ice-Wedge Thermokarst on Banks Island, Remote Sens.-Basel, 10, 954, https://doi.org/10.3390/rs10060954, 2018.
Fraser, T. A. and Burn, C. R.: On the nature and origin of “muck” deposits in the Klondike area, Yukon Territory, Can. J. Earth Sci., 34, 1333–1344, https://doi.org/10.1139/e17-106, 1997.
French, H. M.: The Periglacial Environment Longman, Longman, New York, NY, USA, 1976.
French, H. M.: The Periglacial Environment 4e, 1st edn., Wiley, https://doi.org/10.1002/9781119132820, 2018.
Gooseff, M. N., Balser, A., Bowden, W. B., and Jones, J. B.: Effects of Hillslope Thermokarst in Northern Alaska, Eos T. Am. Geophys. Un., 90, 29–30, https://doi.org/10.1029/2009EO040001, 2009.
Grigoriev, N. F.: Permafrost of the coastal zone of Yakutia, Nauka Publisher: Moscow, Russia, 180 pp., 1966 (in Russian).
Grigoriev, N. F. and Karpov, E. G.: On the origin of the layer of tabular ground ice on the river Yenisei at the latitude of the Arctic Circle, Tabular ground ice of cryolythozone, Yakutsk, Institute of Permafrost Siberian Branch of the USSR Academy of Sciences, 62–71, 1982 (in Russian).
Grom, J. and Pollard, W.: A study of high Arctic retrogressive thaw slump dynamics, Eureka Sound Lowlands, Ellesmere Island, in: Proceedings of the ninth international conference on permafrost, Institute of Northern Engineering, University of Alaska Fairbanks, Alaska 28 June–3 July 2008, 545–550, 2008.
Gubarkov, A., Khomutov, A., and Leibman, M.: Contribution of lateral thermoerosion and thermodenudation to the coastal retreat at Yugorsky Peninsula, Russia, EGU General Assembly Conference Abstracts, 1785, Vienna, Austria, 19–24 April 2009.
Günther, F., Overduin, P. P., Sandakov, A., Grosse, G., and Grigoriev, M. N.: Thermo-erosion along the yedoma coast of the Buor Khaya peninsula, Laptev Sea, east Siberia, in: Proceedings of the Tenth International Conference on Permafrost, Volume 1: International Contributions, Salekhard, Russia, 25–29 June 2012, 137–142, 2012.
Harris, C.: Geomorphological applications of soil micromorphology with particular reference to periglacial sediments and processes, in: Geomorphology and Soils, edited by: Richards, K. S., Arnett, R. R., and Ellis, S., London, UK, Routledge, 219–232, ISBN 9780429320781, 1984.
Harris, C. and Lewkowicz, A. G.: An analysis of the stability of thawing slopes, Ellesmere Island, Nunavut, Canada, Can. Geotech. J., 37, 449–462, https://doi.org/10.1139/t99-118, 2000.
Harry, D. G. and MacInnes, K. L.: The effect of forest fires on permafrost terrain stability, Little Chicago-Travaillant Lake area, Mackenzie Valley, NWT, Current Research, Part D, Ottawa, Geological Survey of Canada, 91–94, 1988.
Highland, L. M. and Bobrowsky, P.: The landslide handbook-A guide to understanding landslides, US Geological Survey, 2008.
Hu, B., Wu, Y., Zhang, X., Yang, B., Chen, J., Li, H., Chen, X., and Chen, Z.: Monitoring the Thaw Slump-Derived Thermokarst in the Qinghai-Tibet Plateau Using Satellite SAR Interferometry, J. Sensors, 2019, 1–8, https://doi.org/10.1155/2019/1698432, 2019.
Huang, L., Liu, L., Jiang, L., and Zhang, T.: Automatic Mapping of Thermokarst Landforms from Remote Sensing Images Using Deep Learning: A Case Study in the Northeastern Tibetan Plateau, Remote Sens.-Basel, 10, 2067, https://doi.org/10.3390/rs10122067, 2018.
Huang, L., Luo, J., Lin, Z., Niu, F., and Liu, L.: Using deep learning to map retrogressive thaw slumps in the Beiluhe region (Tibetan Plateau) from CubeSat images, Remote Sens. Environ., 237, 111534, https://doi.org/10.1016/j.rse.2019.111534, 2020.
Huang, L., Lantz, T. C., Fraser, R. H., Tiampo, K. F., Willis, M. J., and Schaefer, K.: Accuracy, Efficiency, and Transferability of a Deep Learning Model for Mapping Retrogressive Thaw Slumps across the Canadian Arctic, Remote Sens.-Basel, 14, 2747, https://doi.org/10.3390/rs14122747, 2022.
Hughues, O. L.: Surficial geology and land classification, in: Proceedings Canadian Northern Pipeline Conference, Ottawa, Ontario, February 1972, 17–24, 1972.
Ingólfsson, Ó. and Lokrantz, H.: Massive ground ice body of glacial origin at Yugorski Peninsula, arctic Russia, Permafrost Periglac., 14, 199–215, https://doi.org/10.1002/ppp.455, 2003.
Jiao, C., Niu, F., He, P., Ren, L., Luo, J., and Shan, Y.: Deformation and Volumetric Change in a Typical Retrogressive Thaw Slump in Permafrost Regions of the Central Tibetan Plateau, China, Remote Sens.-Basel, 14, 5592, https://doi.org/10.3390/rs14215592, 2022.
Jones, M. K. W., Pollard, W. H., and Jones, B. M.: Rapid initialization of retrogressive thaw slumps in the Canadian high Arctic and their response to climate and terrain factors, Environ. Res. Lett., 14, 055006, https://doi.org/10.1088/1748-9326/ab12fd, 2019.
Jorgenson, M. and Osterkamp, T.: Response of boreal ecosystems to varying modes of permafrost degradation, Can. J. Forest Res., 35, 2100–2111, 2005.
Jorgenson, M. T., Shur, Y. L., and Osterkamp, T. E.: Thermokarst in Alaska, in: Proceedings of the Ninth International Conference on Permafrost, 29 June–3 July 2008, Fairbanks, , Alaska, 869–876, 2008.
Kachurin, S.: Thermokarst on the territory of the USSR, Academy of Sciences Press, Moscow, USSR, 289 pp., 1961 (in Russian).
Kachurin, S.: Thermokarst within the territory of the USSR, Biuletyn Peryglacjalny, 11, 49–55, 1962.
Kachurin, S. P.: Is thermokarst always a sign of permafrost degradation?, Collection: Materials for the fundamentals of the doctrine of frozen zones of the earth's crust, vol. II. M., Publishing House of the USSR Academy of Sciences, Moscow, USSR, 1955 (in Russian).
Kaplina, T. N.: Cryogenic slope processes, Nauka Publisher, Moscow, Russia, 296 pp., 1965 (in Russian).
Kerfoot, D. E.: The geomorphology and permafrost conditions of Garry Island, N. W. T., Text, University of British Columbia, Vancouver, University of British Columbia Library, https://doi.org/10.14288/1.0102152, 1969.
Khomutov, A. and Leibman, M.: Landscape factors of thermodenudation rate at the Yugorsky Peninsula coast, Earth's Cryosphere, 12, 24–35, 2008 (in Russian).
Khomutov, A. and Leibman, M.: Rating of cryogenic translational landsliding hazard in tundra of central Yamal, Kriosfera Zemli, 20, 49–60, 2016.
Kizyakov, A. I.: Dynamics of thermodenudation processes in the area of widespread tabular ground ice occurrence, PhD thesis, Moscow State University, Moscow, Russia, 2005 (in Russian).
Kizyakov, A. I., Leibman, M. O., and Perednya, D. D.: Destructive relief forming processes at the coasts of the arctic plains with tabular ground ice, Earth's Cryosphere, 10, 79–89, 2006 (in Russian).
Kizyakov, A. I., Wetterich, S., Günther, F., Opel, T., Jongejans, L. L., Courtin, J., Meyer, H., Shepelev, A. G., Syromyatnikov, I. I., Fedorov, A. N., Zimin, M. V., and Grosse, G.: Landforms and degradation pattern of the Batagay thaw slump, Northeastern Siberia, Geomorphology, 420, 108501, https://doi.org/10.1016/j.geomorph.2022.108501, 2023.
Kjær, K. H. and Krüger, J.: The final phase of dead-ice moraine development: processes and sediment architecture, Kötlujökull, Iceland, Sedimentology, 48, 935–952, https://doi.org/10.1046/j.1365-3091.2001.00402.x, 2001.
Kokelj, S. V. and Jorgenson, M. T.: Advances in thermokarst research, Permafrost Periglac., 24, 108–119, https://doi.org/10.1002/ppp.1779, 2013.
Kokelj, S. V. and Lewkowicz, A. G.: Salinization of permafrost terrain due to natural geomorphic disturbance, Fosheim Peninsula, Ellesmere Island, Arctic, 52, 325–440, 1999.
Kokelj, S. V., Smith, C. A. S., and Burn, C. R.: Physical and chemical characteristics of the active layer and permafrost, Herschel Island, western Arctic Coast, Canada, Permafrost Periglac., 13, 171–185, https://doi.org/10.1002/ppp.417, 2002.
Kokelj, S. V., Jenkins, R. E., Milburn, D., Burn, C. R., and Snow, N.: The influence of thermokarst disturbance on the water quality of small upland lakes, Mackenzie Delta region, Northwest Territories, Canada, Permafrost Periglac., 16, 343–353, https://doi.org/10.1002/ppp.536, 2005.
Kokelj, S. V., Lantz, T. C., Kanigan, J., Smith, S. L., and Coutts, R.: Origin and polycyclic behaviour of tundra thaw slumps, Mackenzie Delta region, Northwest Territories, Canada, Permafrost Periglac., 20, 173–184, https://doi.org/10.1002/ppp.642, 2009.
Kokelj, S. V., Tunnicliffe, J., Lacelle, D., Lantz, T. C., Chin, K. S., and Fraser, R.: Increased precipitation drives mega slump development and destabilization of ice-rich permafrost terrain, northwestern Canada, Global Planet Change, 129, 56–68, https://doi.org/10.1016/j.gloplacha.2015.02.008, 2015.
Kokelj, S. V., Lantz, T. C., Tunnicliffe, J., Segal, R., and Lacelle, D.: Climate-driven thaw of permafrost preserved glacial landscapes, northwestern Canada, Geology, 45, 371–374, https://doi.org/10.1130/G38626.1, 2017.
Kokelj, S. V., Gingras-Hill, T., Daly, S. V., Morse, P. D., Wolfe, S. A., Rudy, A. C. A., van der Sluijs, J., Weiss, N., Brendan O'Neill, H., Baltzer, J. L., Lantz, T. C., Gibson, C., Cazon, D., Fraser, R. H., Froese, D. G., Giff, G., Klengenberg, C., Lamoureux, S. F., Quinton, W. L., Turetsky, M. R., Chiasson, A., Ferguson, C., Newton, M., Pope, M., Paul, J. A., Wilson, M. A., and Young, J. M.: The Northwest Territories Thermokarst Mapping Collective: a northern-driven mapping collaborative toward understanding the effects of permafrost thaw, Arctic Science, 9, 886–918, https://doi.org/10.1139/as-2023-0009, 2023.
Kotlyakov, V.: Glossary of Glaciology, Hidrometeoizdat, Leningrad, USSR, 1984 (in Russian).
Kudryavcev, V. A.: General permafrost studies (geocryology), 2nd edn., Moscow State University, Moscow, 464 pp., 1978.
Lacelle, D., Bjornson, J., Lauriol, B., Clark, I. D., and Troutet, Y.: Segregated-intrusive ice of subglacial meltwater origin in retrogressive thaw flow headwalls, Richardson Mountains, NWT, Canada, Quaternary Sci. Rev., 23, 681–696, https://doi.org/10.1016/j.quascirev.2003.09.005, 2004.
Lacelle, D., Bjornson, J., and Lauriol, B.: Climatic and geomorphic factors affecting contemporary (1950-2004) activity of retrogressive thaw slumps on the Aklavik Plateau, Richardson Mountains, NWT, Canada, Permafrost Periglac., 21, 1–15, https://doi.org/10.1002/ppp.666, 2010.
Lacelle, D., Brooker, A., Fraser, R. H., and Kokelj, S. V.: Distribution and growth of thaw slumps in the Richardson Mountains–Peel Plateau region, northwestern Canada, Geomorphology, 235, 40–51, https://doi.org/10.1016/j.geomorph.2015.01.024, 2015.
Lamothe, C. and St-Onge, D.: A note on a periglacial erosional process in the Isachsen Area NWT, Geographical bulletin, 16, 104–113, 1961.
Lamoureux, S. F. and Lafrenière, M. J.: Fluvial Impact of Extensive Active Layer Detachments, Cape Bounty, Melville Island, Canada, Arc. Antarct. Alp. Res., 41, 59–68, https://doi.org/10.1657/1523-0430-41.1.59, 2009.
Lantuit, H. and Pollard, W. H.: Temporal stereophotogrammetric analysis of retrogressive thaw slumps on Herschel Island, Yukon Territory, Nat. Hazards Earth Syst. Sci., 5, 413–423, https://doi.org/10.5194/nhess-5-413-2005, 2005.
Lantuit, H. and Pollard, W. H.: Fifty years of coastal erosion and retrogressive thaw slump activity on Herschel Island, southern Beaufort Sea, Yukon Territory, Canada, Geomorphology, 95, 84–102, https://doi.org/10.1016/j.geomorph.2006.07.040, 2008.
Lantuit, H., Pollard, W. H., Couture, N., Fritz, M., Schirrmeister, L., Meyer, H., and Hubberten, H.-W.: Modern and Late Holocene Retrogressive Thaw Slump Activity on the Yukon Coastal Plain and Herschel Island, Yukon Territory, Canada, Permafrost Periglac., 23, 39–51, https://doi.org/10.1002/ppp.1731, 2012.
Lantz, T. C. and Kokelj, S. V.: Increasing rates of retrogressive thaw slump activity in the Mackenzie Delta region, N. W. T., Canada, Geophys. Res. Lett., 35, L06502, https://doi.org/10.1029/2007GL032433, 2008.
Leibman, M.: Cryogenic slope processes and their geoecologic consequences under the tabular ground ice distribution, Doctor of science dissertation, Earth Cryosphere Institute SB RAS, Tyumen, 2005 (in Russian).
Leibman, M., Kizyakov, A., Archegova, I., and Gorlanova, L.: Stages of cryogenic landslide evolution on the Yugorsky Peninsula and Yamal, Earth's Cryosphere, 4, 67–75, 2000 (in Russian).
Leibman, M., Gubarkov, A., Khomutov, A., Kizyakov, A., and Vanshtein, B.: Coastal processes at the tabular-ground-ice-bearing area, Yugorsky Peninsula, Russia, Proceedings of the Ninth International Conference on Permafrost, 28 June–3 July 2008, Fairbanks, USA, 1037–1042, 2008.
Leibman, M., Khomutov, A., and Kizyakov, A.: Cryogenic landslides in the Arctic plains of Russia: Classification, mechanisms, and landforms, in: Landslide Science for a Safer Geoenvironment, edited by: Sassa, K., Canuti, P., and Yin, Y., Springer, 493–497, https://doi.org/10.1007/978-3-319-04996-0_75, 2014.
Leibman, M., Kizyakov, A., Zhdanova, Y., Sonyushkin, A., and Zimin, M.: Coastal Retreat Due to Thermodenudation on the Yugorsky Peninsula, Russia during the Last Decade, Update since 2001–2010, Remote Sens.-Basel, 13, 4042, https://doi.org/10.3390/rs13204042, 2021.
Leibman, M. O.: Cryolithological peculiarities of the active layer on slopes in relation to cryogenic landslides, Earth's Cryosphere, 1, 50–55, 1997 (in Russian).
Leibman, M. O. and Egorov, I. P.: Climatic and environmental controls of cryogenic landslides, Yamal, Russia, in: Landslides: 1941-–1946, Rotterdam, Balkema, ISBN 90-5410-821-5, 1996.
Leibman, M. O. and Kizyakov, A. I.: Cryogenic landslides of the Yamal and Yugorsky Peninsulas, Earth Cryosphere Institute SB RAS, Moscow, 2007 (in Russian).
Leibman, M. O., Kizyakov, A. I., Sulerzhitsky, L. D., and Zaretskaia, N. E.: Dynamics of landslide slopes and their development on Yamal Peninsula, in: Permafrost, Proceedings of the 8th international conference on permafrost, 21–25 July 2003, Zürich, Switzerland, Swets and Zeitlinger, Lisse, 651–656, 2003.
Leibman, M. O., Nesterova, N., and Altukhov, M.: Distribution and Morphometry of Thermocirques in the North of West Siberia, Russia, Geosciences, 13, 167, https://doi.org/10.3390/geosciences13060167, 2023a.
Leibman, M. O., Kizyakov, A. I., Nesterova, N. B., and Tarasevich, I. I.: Classification of cryogenic-landslide landforms for mapping and prediction, Arctic and Antarctic Research, 69, 486–500, 2023b (in Russian).
Lewkowicz, A. G.: Headwall retreat of ground-ice slumps, Banks Island, Northwest Territories, Can. J. Earth Sci., 24, 1077–1085, https://doi.org/10.1139/e87-105, 1987a.
Lewkowicz, A. G.: Nature and Importance of Thermokarst Processes, Sand Hills Moraine, Banks Island, Canada, Geogr. Ann. A, 69, 321–327, https://doi.org/10.1080/04353676.1987.11880218, 1987b.
Lewkowicz, A. G.: Morphology, frequency and magnitude of active-layer detachment slides, Fosheim Peninsula, Ellesmere Island, NWT, Permafrost-Canada, Proceedings of the Fifth Canadian Permafrost Conference, Université Laval, Nordicana, 6 June, 111–118, 1990.
Lewkowicz, A. G.: Dynamics of active-layer detachment failures, Fosheim Peninsula, Ellesmere Island, Nunavut, Canada, Permafrost Periglac., 18, 89–103, https://doi.org/10.1002/ppp.578, 2007.
Lewkowicz, A. G. and Harris, C.: Morphology and geotechnique of active-layer detachment failures in discontinuous and continuous permafrost, northern Canada, Geomorphology, 69, 275–297, https://doi.org/10.1016/j.geomorph.2005.01.011, 2005.
Lewkowicz, A. G. and Way, R. G.: Extremes of summer climate trigger thousands of thermokarst landslides in a High Arctic environment, Nat. Commun., 10, 1329, https://doi.org/10.1038/s41467-019-09314-7, 2019.
Lipovsky, P. and Huscroft, C.: A reconnaissance inventory of permafrost-related landslides in the Pelly River watershed, central Yukon, in: Yukon Exploration and Geology 2006, edited by: Emond, D. S., Lewis, L. L., and Weston, L. H., Yukon Geological Survey, 181–195, 2006.
Mackay, J. R.: Segregated epigenetic ice and slumps in permafrost, Mackenzie Delta area, NWT, Geographical Bulletin, 8, 59–80, 1966.
Mackay, J. R.: Disturbances to the tundra and forest tundra environment of the western Arctic, Can. Geotech. J., 7, 420–432, https://doi.org/10.1139/t70-054, 1970.
Mackay, J. R.: Active layer slope movement in a continuous permafrost environment, Garry Island, Northwest Territories, Canada, Can. J. Earth Sci., 18, 1666–1680, https://doi.org/10.1139/e81-154, 1981. .
Maksimov, V. V.: Results of long-term observations of thermodenudation of career sidewalls in ice complex deposits, in: Methods for studying cryogenic physical and geological processes: Collection of scientific articles, VSEGINGEO, Moscow, Russia, 60–71, 1992 (in Russian).
McRoberts, E. C. and Morgenstern, N. R.: The Stability of Thawing Slopes, Can. Geotech. J., 11, 447–469, https://doi.org/10.1139/t74-052, 1974.
Mesquita, P. S., Wrona, F. J., and Prowse, T. D.: Effects of retrogressive permafrost thaw slumping on sediment chemistry and submerged macrophytes in Arctic tundra lakes, Freshwater Biol., 55, 2347–2358, 2010.
Mukhin, N. I.: To the definition of the term “thermokarst”, Proceedings of the Institute of Permafrost Studies named after VA Obrucheva, 14, 74–81, 1960 (in Russian).
Muller, S. W.: Permafrost of Permanently Frozen Ground and Related Engineering Problems, J. W. Edwards, Inc., Ann Arbor, Michigan, 231 pp., 1947.
Murton, J. B.: Thermokarst sediments and sedimentary structures, Tuktoyaktuk Coastlands, western Arctic Canada, Global Planet. Change, 28, 175–192, https://doi.org/10.1016/S0921-8181(00)00072-2, 2001.
Murton, J. B. and French, H. M.: Thermokarst involutions, summer island, pleistocene mackenzie delta, Western Canadian Arctic, Permafrost Periglac., 4, 217–229, https://doi.org/10.1002/ppp.3430040304, 1993.
Nesterova, N. B., Khomutov, A. V., Leibman, M. O., Safonov, T. A., and Belova, N. G.: The inventory of retrogressive thaw slumps (thermocirques) in the north of West Siberia based on 2016-2018 satellite imagery mosaic, Earth's Cryosphere, 25, 41–50, https://doi.org/10.15372/KZ20210604, 2021.
Nesterova, N. B., Nitze, I., Grosse, G., and Leibman, M. O.: Variability in spectral properties of retrogressive thaw slumps at Vaskiny Dachi research station, central Yamal Peninsula, 6th European Conference on Permafrost (EUCOP 2023), Puigcerdà, Catalonia, Spain, 18–22 June, 2023, 188, 2023.
Nicu, I. C., Lombardo, L., and Rubensdotter, L.: Preliminary assessment of thaw slump hazard to Arctic cultural heritage in Nordenskiöld Land, Svalbard, Landslides, 18, 2935–2947, https://doi.org/10.1007/s10346-021-01684-8, 2021.
Nitze, I., Heidler, K., Barth, S., and Grosse, G.: Developing and Testing a Deep Learning Approach for Mapping Retrogressive Thaw Slumps, Remote Sens.-Basel, 13, 4294, https://doi.org/10.3390/rs13214294, 2021.
Nitze, I., Van der Sluijs, J., Barth, S., Bernhard, P., Huang, L., Kizyakov, A., Lara, M., Nesterova, N., Runge, A., Veremeeva, A., Ward Jones, M., Witharana, C., Xia, Z., and Liljedahl, A.: A Labeling Intercomparison of Retrogressive Thaw Slumps by a Diverse Group of Domain Experts, Permafrost Periglac., https://doi.org/10.1002/ppp.2249, 2024.
Niu, F., Luo, J., Lin, Z., Ma, W., and Lu, J.: Development and thermal regime of a thaw slump in the Qinghai–Tibet plateau, Cold Reg. Sci. Technol., 83–84, 131–138, https://doi.org/10.1016/j.coldregions.2012.07.007, 2012.
Niu, F., Luo, J., Lin, Z., Fang, J., and Liu, M.: Thaw-induced slope failures and stability analyses in permafrost regions of the Qinghai-Tibet Plateau, China, Landslides, 13, 55–65, https://doi.org/10.1007/s10346-014-0545-2, 2016.
Panov, D. G.: Denudation zones of Arctic Eurasia, in: Issues of physical geography, vol. 3, Publishing house of the USSR Academy of Sciences, Moscow – Leningrad, USSR, 139–143, 1936 (in Russian).
Péwé, T. L.: Effect of permafrost on cultivated fields, Fairbanks area, Alaska, Geological Survey Bulletin, 315–351, https://doi.org/10.3133/b989F, 1954.
Pizhankova, E.: Termodenudation in the coastal zone of the Lyakhovsky islands (interpretation of aerospace images), Earth's Cryosphere, 15, 61–70, 2011 (in Russian).
Pollard, W. H.: Distribution and characterization of ground ice on Fosheim Peninsula, Ellesmere Island, Nunavut, Geological Survey of Canada, Bulletin, 529, 207–233, https://doi.org/10.4095/211959, 2000.
Popov, A.: Le thermokarst (L'érosion thermique actuelle et ses traces figées dans le paysage de la Pologne), Biuletyn peryglacjalny, 4, 319–330, 1956.
Popov, A. I.: Permafrost phenomena in the earth's crust: (Cryolithology): Textbook, Moscow State University, Moscow, USSR, 303 pp., 1967 (in Russian).
Popov, A. I., Kachurin, S. P., and Grave, N. A.: Features of the development of frozen geomorphology in northern Eurasia, in: Proceedings of the First International Conference on Permafrost, Washington, DC, 11–15 November 1963, 181–185, 1966.
Poppe, V. and Brown, R. J. E.: Russian-English glossary of permafrost terms, National Research Council of Canada, Associate Committee on Geotechnical Research, https://doi.org/10.4224/20386605, 1976.
Price, L. W.: Subsurface Movement on Solifluction Slopes in the Ruby Range, Yukon Territory, Canada – A 20-Year Study, Arctic Alpine Res., 23, 200–205, https://doi.org/10.1080/00040851.1991.12002837, 1991.
Ramage, J. L., Irrgang, A. M., Herzschuh, U., Morgenstern, A., Couture, N., and Lantuit, H.: Terrain controls on the occurrence of coastal retrogressive thaw slumps along the Yukon Coast, Canada, J. Geophys. Res.-Earth, 122, 1619–1634, https://doi.org/10.1002/2017JF004231, 2017.
Ramage, J. L., Fortier, D., Hugelius, G., Lantuit, H., and Morgenstern, A.: Distribution of carbon and nitrogen along hillslopes in three valleys on Herschel Island, Yukon Territory, Canada, CATENA, 178, 132–140, https://doi.org/10.1016/j.catena.2019.02.029, 2019.
Rampton, V. and Mackay, J. R.: Massive Ice and Icy Sediments Throughout the Tuktoyaktuk Peninsula, Richards Island: And Nearby Areas, District of Mackenzie, Department of Energy, Mines and Resources, 1971.
Robinson, S. D.: Thaw-slump-derived thermokarst near Hot Weather Creek, Ellesmere Island, Nunavut, in: Environmental response to climate change in the Canadian high Arctic, vol. 529, Geological Survey of Canada, Bulletin, Ottawa, 529, 335–345, 2000.
Romanenko, F. A.: Ground ice and relief evolution on the Islands and Coasts of the Russian Arctic, Seventh International Conference on Permafrost, Yellowknife, Canada, 955–959, 1998.
Romanovskii, N., Gravis, G., Melnikov, E., and Leibman, M. O.: Periglacial processes as geoindicators in the cryolithozone, in: Geoindicators: Assessing rapid environmental changes in earth systems, edited by: Berger, A. R., and Iams, W. J., Assessing rapid environmental changes in earth systems, 23–27 June 1998, A.A. Balkema, Brookfield, 47–68, ISBN 90-5410-631-X, 1996.
Romanovskii, N. N.: Foundations of Lithosphere Cryogenesis, Moscow State University, Moscow, 336 pp., 1993 (in Russian).
Rudy, A. C. A., Lamoureux, S. F., Treitz, P., and van Ewijk, K. Y.: Transferability of regional permafrost disturbance susceptibility modelling using generalized linear and generalized additive models, Geomorphology, 264, 95–108, https://doi.org/10.1016/j.geomorph.2016.04.011, 2016.
Runge, A., Nitze, I., and Grosse, G.: Remote sensing annual dynamics of rapid permafrost thaw disturbances with LandTrendr, Remote Sens. Environ., 268, 112752, https://doi.org/10.1016/j.rse.2021.112752, 2022.
Segal, R. A., Lantz, T. C., and Kokelj, S. V.: Acceleration of thaw slump activity in glaciated landscapes of the Western Canadian Arctic, Environ. Res. Lett., 11, 34025, https://doi.org/10.1088/1748-9326/11/3/034025, 2016.
Séjourné, A., Séjourné, A., Costard, F., Fedorov, A., Gargani, J., Skorve, J., Masse, M., Masse, M., and Mège, D.: Evolution of the banks of thermokarst lakes in Central Yakutia (Central Siberia) due to retrogressive thaw slump activity controlled by insolation, Geomorphology, 241, 31–40, 2015.
Shur, Y. U.: Thermokarst (to the thermophysical foundations of the doctrine of the laws of the development of the process), Nedra, Moscow, 80, 1977 (in Russian).
Smith, D. J.: Rates and controls of soil movement on a solifluction slope in the Mount Rae area, Canadian Rocky Mountains, Z. Geomorphol. NF, 71, 25–44, 1988.
Smith, S. L., O'Neill, H. B., Isaksen, K., Noetzli, J., and Romanovsky, V. E.: The changing thermal state of permafrost, Nature Reviews Earth & Environment, 3, 10–23, https://doi.org/10.1038/s43017-021-00240-1, 2022.
Strauss, J., Laboor, S., Schirrmeister, L., Fedorov, A. N., Fortier, D., Froese, D., Fuchs, M., Günther, F., Grigoriev, M., Harden, J., Hugelius, G., Jongejans, L. L., Kanevskiy, M., Kholodov, A., Kunitsky, V., Kraev, G., Lozhkin, A., Rivkina, E., Shur, Y., Siegert, C., Spektor, V., Streletskaya, I., Ulrich, M., Vartanyan, S., Veremeeva, A., Anthony, K. W., Wetterich, S., Zimov, N., and Grosse, G.: Circum-Arctic Map of the Yedoma Permafrost Domain, Frontiers in Earth Science, 9, 758360, https://doi.org/10.3389/feart.2021.758360, 2021.
Sumgin, M., Kachurin, S., Tolstikhin, N., and Tumel, V.: General Permafrost Science, Akademiyia Nauk SSR, Moscow, 1940 (in Russian).
Sun, Z., Wang, Y., Sun, Y., Niu, F., GuoyuLi, and Gao, Z.: Creep characteristics and process analyses of a thaw slump in the permafrost region of the Qinghai-Tibet Plateau, China, Geomorphology, 293, 1–10, https://doi.org/10.1016/j.geomorph.2017.04.045, 2017.
Swanson, D. K. and Hill, K.: Monitoring of retrogressive thaw slumps in the Arctic Network, 2010 baseline data: Three-dimensional modeling with small-format aerial photographs, Natural Resource Data Series NPS/ARCN/NRDS—2010/123, National Park Service, Fort Collins, Colorado, https://doi.org/10.13140/RG.2.2.23389.00489, 2010.
Swanson, D. K.: Permafrost thaw-related slope failures in Alaska's Arctic National Parks, c. 1980–2019, Permafrost Periglac., 32, 392–406, https://doi.org/10.1002/ppp.2098 2021.
Swanson, D. K. and Nolan, M.: Growth of retrogressive thaw slumps in the Noatak Valley, Alaska, 2010–2016, measured by airborne photogrammetry, Remote Sens.-Basel, 10, 983, https://doi.org/10.3390/rs10070983, 2018.
Tikhomirov, B. A.: Some issues of the dynamics of the surface formations of the Arctic in connection with the genesis of the Baydzherakhs mounds, Issues of physical geography, 285, 1958 (in Russian).
Timofeev, D. A. and Vtyurina, E. A.: Terminology of periglacial geomorphology: Materials on geomorphological terminology, Nauka Publisher, Moscow, 231 pp., 1983 (in Russian).
Troll, C.: The subnival or periglacial cycle of denudation, Erdkunde, 2, 1–21, 1948 (in German).
Vaikmäe, R., Michel, F. A., and Solomatin, V. I.: Morphology, stratigraphy and oxygen isotope composition of fossil glacier ice at Ledyanaya Gora, Northwest Siberia, Russia, Boreas, 22, 205–213, https://doi.org/10.1111/j.1502-3885.1993.tb00180.x, 1993.
van der Sluijs, J., Kokelj, S. V., and Tunnicliffe, J. F.: Allometric scaling of retrogressive thaw slumps, The Cryosphere, 17, 4511–4533, https://doi.org/10.5194/tc-17-4511-2023, 2023.
Van Everdingen, R.: Multi-language glossary of permafrost and related ground-ice terms, National Snow and Ice Data Center/World Data Center for Glaciology, Boulder, CO, USA, 2005.
Voskresenskii, K. and Sovershaev, V.: Role of exogenous processes in the dynamics of Arctic coasts, Dynamics of the Arctic Coasts of Russia, Moscow University Press, Moscow, Russia, 35–48, 1998 (in Russian).
Voskresenskii, K. S.: The modern relief-forming processes on plains of the northern Russia, Moscow State University, Moscow, 264, 2001 (in Russian).
Wang, B., Paudel, B., and Li, H.: Retrogression characteristics of landslides in fine-grained permafrost soils, Mackenzie Valley, Canada, Landslides, 6, 121–127, 2009.
Washburn, A. L.: Geocryology: a survey of periglacial processes and environments, 2nd Edn., Edward Arnold (Publishers) Ltd, London, UK, 406 pp., ISBN 0713161191, 1979.
Wei, M., Fujun, N., Satoshi, A., and Dewu, J.: Slope instability phenomena in permafrost regions of Qinghai-Tibet Plateau, China, Landslides, 3, 260–264, https://doi.org/10.1007/s10346-006-0045-0, 2006.
Wolfe, S. A., Kotler, E., and Dallimore, S. R.: Surficial characteristics and the distribution of thaw landforms (1970 to 1999), Shingle Point to Kay Point, Yukon Territory, Open File, 4088, Geological Survey of Canada, 2001.
Worsley, P.: Context of relict Wisconsinan glacial ice at Angus Lake, SW Banks Island, western Canadian Arctic and stratigraphic implications, Boreas, 28, 543–550, https://doi.org/10.1111/j.1502-3885.1999.tb00240.x, 1999.
Yang, D., Qiu, H., Ye, B., Liu, Y., Zhang, J., and Zhu, Y.: Distribution and Recurrence of Warming-Induced Retrogressive Thaw Slumps on the Central Qinghai-Tibet Plateau, J. Geophys. Res.-Earth, 128, e2022JF007047, https://doi.org/10.1029/2022JF007047, 2023.
Yershov, E. D.: General Geocryology, edited by: Williams, P. J., Cambridge University Press, Cambridge, https://doi.org/10.1017/CBO9780511564505, 1998.
Zenkovich, V. P. and Popov, B. A.: Marine geomorphology. Terminological reference book. Coastal zone: processes, concepts, definitions, Publisher Mysl', Moscow, USSR, 280 pp., 1980 (in Russian).
Zhigarev, L. A.: Termodenudation Processes and Deformation Behavior of the Thawing Ground, Nauka Publisher: Moscow, Russia, 110 pp., 1975 (in Russian).
Zhigarev, L. A.: Post-cryogenic soil flows on slopes and coastal cliffs, in: General permafrost science: Proceedings of the III International Conference on Permafrost Science, 10–13 July 1978, Edmonton, Alberta, Canada 141–151, 1978 (in Russian).
Zoltai, S. C. and Pettapiece, W. W.: Studies of vegetation, landform and permafrost in the Mackenzie Valley: Terrain, vegetation and permafrost relationships in the northern part of the Mackenzie Valley and northern Yukon, Environmental-Social Committee Northern Pipelines, Task Force on Northern Oil Development, 1973.
Zwieback, S., Kokelj, S. V., Günther, F., Boike, J., Grosse, G., and Hajnsek, I.: Sub-seasonal thaw slump mass wasting is not consistently energy limited at the landscape scale, The Cryosphere, 12, 549–564, https://doi.org/10.5194/tc-12-549-2018, 2018.
Zwieback, S., Boike, J., Marsh, P., and Berg, A.: Debris cover on thaw slumps and its insulative role in a warming climate, Earth Surf. Proc. Land., 45, 2631–2646, https://doi.org/10.1002/esp.4919, 2020.